Micro electromechanical systems (mems) based microfluidic devices for biomedical applications
Int. J. Mol. Sci. 2011,
12, 3648-3704; doi:10.3390/ijms12063648
OPEN ACCESS
International Journal of
Molecular Sciences
ISSN 1422-0067
Micro Electromechanical Systems (MEMS) Based Microfluidic
Devices for Biomedical Applications
Muhammad Waseem Ashraf *, Shahzadi Tayyaba and Nitin Afzulpurkar
School of Engineering and Technology, Asian Institute of Technology (AIT), Bangkok 12120,
Thailand; E-Mails:
[email protected] (S.T.);
[email protected] (N.A.)
* Author to whom correspondence should be addressed;
E-Mail:
[email protected]; Tel.: +66-8-73516061; Fax: +025245697.
Received: 10 April 2011; in revised form: 3 May 2011 / Accepted: 19 May 2011 /
Published: 7 June 2011
Abstract: Micro Electromechanical Systems (MEMS) based microfluidic devices have
gained popularity in biomedicine field over the last few years. In this paper, a
comprehensive overview of microfluidic devices such as micropumps and microneedles
has been presented for biomedical applications. The aim of this paper is to present the
major features and issues related to micropumps and microneedles, e.g., working
principles, actuation methods, fabrication techniques, construction, performance
parameters, failure analysis, testing, safety issues, applications, commercialization issues
and future prospects. Based on the actuation mechanisms, the micropumps are classified
into two main types,
i.e., mechanical and non-mechanical micropumps. Microneedles can
be categorized according to their structure, fabrication process, material, overall shape, tip
shape, size, array density and application. The presented literature review on micropumps
and microneedles will provide comprehensive information for researchers working on
design and development of microfluidic devices for biomedical applications.
Keywords: drug delivery system; microfluidics; micropumps; microneedles
1. Introduction
Microfluidics is a relatively new branch of science and technology which has made extensive
progress in the last few years. Microfluidic systems deal with the fluid flow in diminutive amounts,
Int. J. Mol. Sci. 2011,
12
typically a few microlitres (μL) in a miniaturized system. The main functions performed by these
systems are sample preparation, purification, separation, reaction, transport, immobilization, labeling,
biosensing and detection. Fluid behavior at macro scale is quite different from micro and nano scale.
Factors such as surface tension may become dominant in microfluidic devices. When the size of
biological samples is close to the flow channels or needles through which the samples are transported,
then the sample flow may not be envisaged on the basis of conventional fluidic systems. Considerable
research has been made in recent years in the field of microfluidic components, devices, systems and
fabrication methods. The use of micro and nano electromechanical systems (MEMS and NEMS)
technology has been increasing rapidly to fabricate microfluidic devices for biomedical applications.
Due to MEMS and NEMS technology, the fabrication of miniature size and high performance medical
devices has become practicable to congregate the critical medical requirements like controlled delivery
with negligible side effects, improved bioavailability and therapeutic effectiveness [1,2]. In recent
years, the most important advancement of MEMS and NEMS in biomedicine is microfluidic
transdermal drug delivery (TDD) systems [3]. TDD systems deal with the movement of
pharmaceutical compound through the skin to reach the systemic circulation for subsequent
distribution in the human body [4]. TDD system consists of micropumps, microneedles, reservoir,
micro-flow sensor, blood pressure sensor, and required electronic circuit for necessary operations.
Among them, micropumps and microneedles are the most important components of microfluidic
system particularly for drug delivery applications. Micropumps are used for delivery and treatment
purposes. Microneedles can be used as stand-alone devices and part of complicated microfluidic
system in which microneedles are integrated with other devices in the system. The schematic
illustration of transdermal drug delivery system is shown in Figure 1.
Figure 1. Schematic illustration of transdermal drug delivery (TDD) system.
Int. J. Mol. Sci. 2011,
12
In recent years, a few TDD products have been reported and approved by the US FDA. IONSYS
(Fentanyl ionophoretic), a product by Alza Corporation was approved in 2006 for patient controlled
pain management. Emsam, a product by Bristol-Myers Squibb (Princeton, NJ, USA) was approved in
2006 for major depressive disorder. Fentanyl generic by Watson Pharmaceuticals was approved in
2007 as an analgesic. Neupro, by Schwarz Pharma (Mequon, WI, USA) was approved in 2007 for
Parkinson's disease. Exelon, by Novartis (East Hannover, NJ, USA) was approved in 2007 for
dementia [5]. Similarly various researchers have presented microfluidic devices for different medical
applications. Particularly micropumps and microneedles have been extensively studied in this decade
for biomedicine. But there is still a need to present the latest updates on the development of micropumps
and microneedles for biomedicine because these devices are still at the research level and have limited
availability for commercial use. Some earlier reviews on various applications of MEMS in the
biomedical field have been reported, such as the therapeutic microsystem, surgical microsystem and drug
therapy. These reviews provide basic information on various devices such as microneedles, micropumps,
micro-reservoirs,
etc. [6–9]. Various researchers have reported reviews on design and development of
micropumps only [10–14]. Laser and Santiago [10] presented a comprehensive review on micropumps.
But the review did not cover some actuation methods, e.g., ion conductive polymer film (ICPF),
development of evaporation micropump and advance applications of micropumps in biomedicine.
Woias [11] presented a concise overview of different types of micropumps and their applications.
However, the electrowetting micropump, evaporation micropump and ICPF have not been described in
the review. Tsai and Sue [12] reported introductory overview on the importance of micropumps for
medical applications, but significant details about the applications of various kinds of micropumps for
drug delivery have not been presented. Nisar
et al. [13] presented a comprehensive and good review on
various types of micropumps and their applications in biomedical applications. Some key features of
micropumps like actuation techniques, performance parameters, working principles, structure,
fabrication and applications have been reported, but this review has not covered latest developments in
micropumps for biomedical applications. The review does not provide up-to-date information about
bio-MEMS devices as there is an exponential increase in design and development in the bio-medicine
field. Amirouche
et al. [14] presented a review on current developments in micropumps. The focus of
this review was on mechanical micropumps and their applications in the biomedical field. However
this review has not covered non-mechanical type of micropumps. Grayson
et al. [15] reported a brief
review on various integrated MEMS devices such as biosensors, stents, immunoisolation devices,
reservoirs, microneedles,
etc. This review has not described all parameters of MEMS devices like
design, development, actuation methods, fabrication techniques,
etc. Karman
et al. [16] reported a very
basic and introductory review on drug delivery devices like micropumps, microneedles, microvalves,
microactuators, microreservoirs,
etc. This review has not covered important parameters such as
actuation techniques, working principles, performance constraints, design, fabrication and applications
of MEMS devices. Bao-jian
et al. [17] presented information on the development and applications of
MEMS based microneedles. This review has not covered some important aspects of design and
development, forces experienced by microneedles, testing, structural/fluidic analyses,
etc.
Khanna
et al. [18] reported a review on the particular design requirements of microneedles for diabetic
therapy. This review has not covered the key parameters like development, fabrication, failure
analysis,
etc. Sachdeva and Banga [19] reported good comprehensive review on microneedles design,
Int. J. Mol. Sci. 2011,
12
development, safety and regulatory issue, therapeutic applications and limitations of microneedles for
commercialization. However, this review has not described the fabrication techniques of microneedles,
failure of microneedles due to various applied forces, structural and fluidic analysis and integration
issues of microneedles with micropumps. All reviews that have been discussed above present the
information about micropumps or microneedles only. Here the authors have presented a review on
micropumps and microneedles that covers most recent advancement of MEMS technology in
biomedicine. This is the first comprehensive and updated review that covers latest information of
microfluidic devices regarding the design, development, actuation methods, performance parameters,
working principles, structure, fabrication techniques, material used for fabrication, safety issue,
challenges, limitations of commercialization and applications. This comprehensive review will be
helpful for researchers who would like to work in the fast growing field of bio-MEMS and bio-NEMS.
2. Micropumps
Pioneering work on micropumps started in the 1970s and developments based on microfabrication
technology was initiated in the 1980s. The MEMS based micropump was developed in 1990s. The
micropump is the main component of drug delivery system that provides the actuation mechanism to
deliver specific volumes of therapeutic agents/drugs from the reservoir. The requirements for drug
delivery include a minimum flow rate in order of 10 µL per minute or more, small size and high
reliability [13]. Normally a micropump consists of the following components: diaphragm membrane,
chamber, actuator, microchannels, microvalves, inlet, outlet,
etc. Micropumps can be categorized into
two classes: One type has a mechanical moving part and is known as a mechanical micropump; the
other has no moving part and is known as a non-mechanical micropump.
2.1. Design Specifications and Parameters of Micropumps
Design of micropumps plays an important role for practical applications of devices. To develop a
suitable design of micropumps for real time applications, it is very important to understand terms like
actuator, valves, chamber or reservoir, nozzle diffuser mechanism and pumping parameters properly.
The actuator is the necessary and driving part of a micropump that converts energy into motion. It is
used to provide force for fluid flow in micropumps. The actuator takes energy from electricity, heat,
liquid pressure, air pressure and converts it into some kind of motion. In most micropumps reported in
literature, the actuation disk is attached with membrane which is used to push the fluid. Some types of
time diaphragm are fabricated in such a way that it produces energy itself which pushes the fluid. In
peristaltic micropumps more than one actuator is fabricated sequentially.
In micropumps, valves are used to control the flby opening, closing and partially hindering
passageways. In microfluidic systems, active and passive valves have been reported. In passive valves
there is no actuation mechanism. The control of fluid flow is dependent on the pressure difference in
Int. J. Mol. Sci. 2011,
12
liquid chamber and the fluid flow is normally in one direction. In active valves, active elements are
present for opening and closing that are operated by an external actuation source. Mostly, separate
components have been reported for active micro-valves for regulating the fluid flow in microfluidic
systems. It is very easy to control the active valves but they are more complicated in integrated
microfluidic system.
2.1.3. Chamber or Reservoir
Chamber design is very critical in microfluidic systems and it can significantly influence the
volume stroke, pressure characteristics and nozzle-diffuser loss coefficients. Most of the micropumps
reported in literature have a single chamber configuration. But in order to improve the performance,
two or three chamber micropumps have also been reported. Micropumps in which pumping chambers
are arranged sequentially or fabricated in such a way that the multiple chambers are in series or in
parallel arrangements, are known as peristaltic micropumps.
2.1.4. Nozzle/Diffuser Element
Nozzle/diffuser element is mostly used in valveless micropumps as a flow rectifier. A schematic
illustration of the nozzle/diffuser action in micropumps is shown in Figure 2. Nozzle/diffuser element
works in such a way that during supply mode more fluid enters in the chamber through an inlet than
fluid that exiting the outlet. While in pump mode the reverse action occurs. Stemme and Stemme [20]
were the first to report valveless miniature micropumps in which they used a nozzle/diffuser element
as flow rectifying element.
Figure 2. Schematic of nozzle/diffuser element.
2.1.5. Pumping Parameters
Various design parameters are important to optimize the performance of micropumps such as
maximum flow rate (Qmax), pump power (Ppump), maximum back pressure (hmax) and pump efficiency (η). Qmax is highest at zero hmax and Qmax is zero when highest value of hmax. For incompressible flow,
the pump head (h) can be calculated from the steady flow energy equation [21].
Int. J. Mol. Sci. 2011,
12
Where, is pressure, is pressure head, is velocity head and is elevation.
The pump efficiency in the form of power can be expressed as:
Ideally, losses are zero and both quantities and are identical. Efficiency is governed
by frictional losses, fluid leakage losses and losses due to imperfect pump construction. The total
efficiency can be expressed as [21].
Where, is mechanical efficiency, is volumetric efficiency and is hydraulic efficiency.
2.2. Mechanical Micropumps
The mechanical micropumps have moving parts so require a physical actuator for the pumping
process. The most common mechanical micropumps are displacement type micropumps that involve a
pumping chamber which is closed with a flexible diaphragm. The fluid flow is achieved by the
oscillation of a diaphragm. Due to these oscillations, the pressure ( P) is created. This pressure is a
function of stroke volume inside the chamber produced by the actuator. The actuator has to run
itself with the dead volume in chamber. Compression ratio is the important parameter for mechanical diaphragm type micropumps. The compression ratio is defined by the equation (4):
The performance of mechanical micropump is normally limited by its mechanical components. The
piezoelectric, electrostatic, thermopneumatic, electromagnetic, bimetallic, ion conductive polymer
films (ICPF), phase change and shape memory alloy (SMA) are examples of mechanical micropumps.
A detailed description of mechanical micropumps is given below.
2.2.1. Piezoelectric Micropumps
The conversion of mechanical energy to electronic signal (voltage) and
vice versa is known as the
piezoelectric effect. The materials which exhibit piezoelectric effect normally have no center of
symmetry in their structure. A stress applied to such materials will alter the separation between the
positive and negative charges that leads to the net polarization at the surface. An electrical field with
voltage potential is created in those materials due to the polarization. This property can be used to form
the actuator, micropump, inkjet printer head,
etc. The effectiveness of energy and
vice versa can be
expressed by factor :
Int. J. Mol. Sci. 2011,
12
Piezoelectric actuator shows large actuation and fast response time, but the fabrication of such
materials is complicated on a single chip. Piezoelectric micropumps exhibit small stroke volume at
high voltages. A schematic of a piezoelectric micropump is shown in Figure 3.
Figure 3. Piezoelectric micropump.
The first piezoelectric micropump was fabricated using micromachining technology by
Van Lintel
et al. [22]. The micropump consisted of a pumping chamber, passive silicon (Si) check
valve, and a thin glass membrane actuated by piezo disk. The maximum flow rate of 8 μL/min and
back pressure of 9.8 kPa were observed at applied 125 V with 1 Hz frequency. Esashi
et al. [23]
reported a three layers piezoelectric pump with flow rate of 15 μL/min and back pressure of 6.4 kPa at
applied 90 V with 30 Hz frequency. Olsson
et al. [24] reported a two chamber piezoelectric
micropump to improve the performance. Koch
et al. [25] presented piezoelectric micropump based on
screen printing of PZT (Lead Zirconate Titanate) on Si membrane. The flow rate of 120 μL/min and
back pressure 2 kPa were observed at applied 600 V with 200 Hz frequency. Schabmueller
et al. [26]
fabricated piezoelectric micropump with passive valves. The flow rate of 1500 μL/min and back
pressure of 1 kPa were achieved using ethanol. Feng and Kim [27] reported piezoelectric micropump that consisted of one way parylene valves. The flow rate of 3.2 μL/min and back pressure of 0.2 kPa were observed at applied 80 V with lower power consumption of 3mW. Geipel
et al. [28] reported a
novel design of micropump with back flow pressure independent flow rate. The back pressure
independency was reported up to 20 kPa at low frequency. Trenkle
et al. [29] reported a piezostack
actuated peristaltic micropump. The flow rate of 40 μL/min was obtained at the frequency of 28.6 Hz
using water. The flow rates were observed to be independent of backpressure up to 7 kPa, with a
maximum backpressure of 45 kPa at 140 V. Johari
et al. [30] reported the fabrication of a piezoelectric
micropump for drug delivery system using two optical masks. Fluidic characteristics analysis was
performed using CoventorWare simulator. Wang
et al. [31] studied the effect of longitudinal flow
asymmetry on pumping capability by using a simple pumping system comprised of a piezoelectric
buzzer imbedded in a channel. Ali
et al. [32] studied the dynamic piezoelectric micropump process.
The quantitative measurement of the pressure generated, applied electrical field, frequency and length
of the actuator, were observed. Liu
et al. [33] proposed a disposable high performance piezoelectric
micropump with four chambers in serial connection for closed loop insulin therapy system. Outflow
resolution of 6.23 × 10 5 mL/pulse was observed. The maximum backpressure of 22 kPa was reported
at applied voltage of 36 Vpp and 200 Hz frequency.
Int. J. Mol. Sci. 2011,
12
2.2.2. Electrostatic Micropumps
Electrostatic micropumps involve electrostatic forces for actuation mechanism. Electrostatic force
is defined as ―the electrical force of attraction and repulsion induced by an electric field ‖. The like charges repel each other and unlike charges attract each others. The electrostatic force applied on the
electrostatic plates can be expressed by the equation (6):
Where, is electrostatic attraction force, is energy stored, is dielectric constant, is area of
electrodes, is electrode spacing and is applied voltage.
Electrostatic actuation is widely used in microfluidic devices. The fabrication of such mechanisms
on electronic chip is very easy, but electrostatic actuator has only a small stroke, typically 10 μm. The
main advantages of electrostatic micropump are low power consumption and fast time response. The
schematic of an electrostatic micropump is shown by Figure 4.
Figure 4. Electrostatic micropump.
The first electrostatic micropump was fabricated by Judy
et al. [34] using surface micromachining
technology. It consisted of active check valve, chamber and active outlet valve. Pumping results were
not reported. The first experimental results of electrostatic micropump were reported by
Zengerle
et al. [35]. The flow rate of 70 μL/min and back pressure of 2.5 kPa were observed at applied
170 V with frequency 25 Hz. Cabuz
et al. [36] presented dual diaphragm electrostatic micropump
using injection molding technique. Micropump was capable of bidirectional operation but only used for gases. The flow rate of 30 μL/min was observed at applied 160 V with frequency of 30 Hz and power of 8 mW. Machauf
et al. [37] presented membrane based electrostatically actuated micropump
across the working fluid. The concept was based on high and low electric permittivity of working fluid.
This pump was limited only for conducting fluid. The flow rate of 1 μL/min was achieved at 50 V.
Astle
et al. [38] proposed a pumping mechanism using electrostatic actuation for gas chromatograph
applications. The flow rate of 3 mL/min and backpressure of 7 kPa were observed at frequency of
14 kHz. Lee
et al. [39] fabricated and tested a peristaltic electrostatic gas micropump that employed
fluidic resonance for high flow rate and multi stage peristaltic configuration. The micropump presented
the pressure ranges from 7.3 to 3.3 kPa and flow rates from 0.29 to 0.07 sccm at the duration time
Int. J. Mol. Sci. 2011,
12
ranges from 0.05 and 0.35 cycles for opening of valves. Liu [40] reported the ―pull in phenomena‖ in
electrostatic micropump using reduced order model of membrane. Various parameters like radius,
thickness, initial gap, residual stress on pull in voltage and pull in position were investigated.
Lil
et al. [41] presented the modeling of micropump membrane with electrostatic actuator. MATLAB
platform was used for modeling. The resonant frequency of 635 Hz for silicon electrostatic actuating
membrane was calculated. Using FEM, 680 Hz frequency was reported.
2.2.3. Thermopneuamtic Micropumps
In thermopneumatic micropumps, the actuator is based on thermal expansion. The chamber is full
of air and thermopneumatic micropump is expanded and compressed periodically by the heater and
cooler. The periodic change in volume of chamber provides the membrane with a regular momentum
that results in fluid out flow. The pressure increase is expressed by the equation (7).
Where, is pressure change, is temperature change, is thermal expansion, is a percentage of
The thermopneumatic type of micropump generates relatively strong pressure and displacement of
membrane. However, the driving power has to be constantly maintained above a certain level. The
schematic diagram of thermopneumatic micropump is shown by the Figure 5.
Figure 5. Thermopneumatic micropump.
The first thermopneumatic micropump based on microfabrication was proposed by
Van De Pol
et al. [42]. The flow rate of 34 μL/min was observed at applied voltage of 6 V with
temperature around 30 ˚C. Jeong and Yang [43] reported a thermopneumatic micropump with corrugated diaphragm. The flow rate of 14 μL/min was observed at applied voltage of 8 V with frequency of 4 Hz. A thermopneumatic micropump consisting of a thin film heater, flow strictor and
two reservoirs has been proposed by Cooney and Towe [44]. The maximum flow rate of 1.4 μL/min
for 4.5 h was observed with an average power of 200 mW. Kim
et al. [45] proposed a
thermopneumatic micropump with a glass layer, indium tin oxide heater, polydimethylsiloxane (PDMS) chamber, PDMS membrane and PDMS cavity. The flow rate of 0.078 μL/min was achieved at applied voltage of 55 V with frequency of 6 Hz. Jeong and Konishi [46] fabricated a peristaltic
Int. J. Mol. Sci. 2011,
12
micropump consisting of three cascaded thermopneumatic actuators and microfluidic channel
connecting two fluidic inlet/outlet ports. The flow rate of 73.9 μL/min was achieved for the de-ionized
(DI) water at zero backpressure. Chia
et al. [47] proposed a novel thermopneumatic peristaltic
micropump comprised of two separate zones for air heating and fluid squeezing. The temperature
elevation of 2.0 K was reported on the fluid pumping area. Tan
et al. [48] fabricated a peristaltic
(polymethylmethacrylate) part where PDMS/adhesive membrane worked like a pneumatic actuator.
The maximum flow rate of 96l μL/min was achieved.
2.2.4. Electromagnetic Micropumps
Electromagnet is a kind of magnet that is based on the combination of electric and magnetic fields.
When the current passes through the coils the magnetic field is produced. The strength of
electromagnet can be easily varied by changing the electric current flowing through the coils. The
force experienced by the point charge due to the electromagnetic field is known as the Lorentz force.
The Lorentz force can be expressed by equation (8).
Where, is force and is magnetic field.
Electromagnetic actuation is large and covers a longer distance as compared to electrostatic
actuation. It needs low voltage but an external source is required for actuation such as a permanent
magnet. On small scale, this type of actuation has no benefit because it is reduced by the cube of
scaling factor. The driving coils or permanent magnets bond directly with the membrane and provide a
magnetic field. However, at the same time, the size is compromised. Usually electromagnetic
micropumps have high power consumption and heat dissipation. A schematic of an electromagnetic
micropump is shown in Figure 6.
Figure 6. Electromagnetic micropump.
The first electromagnetic micropump with 7 μm thick Ni80Fe20 film electroplated on 17 μm thick Si
membrane was proposed by Zheng and Ahn [49]. The maximum flow rate of 20 μL/min was observed
at applied voltage of 3 V with 5 Hz frequency and 300 mA induced current. A plastic micropump with
electromagnetic actuation has been reported by Bohm
et al. [50] that consisted of two folded valves
with a thin membrane in center, inlet/outlet at bottom and pump membrane at top. The maximum flow
Int. J. Mol. Sci. 2011,
12
rates of 40,000 μL/min for air and 2100 μL/min for water were observed with power consumption of
0.5 W. A four layer electromagnetic micropump was designed and its static/dynamic properties were
investigated by Gong
et al. [51]. The membrane deflection by different magnetic driving forces was
analyzed by ANSYS FEM. The maximum flow rate of 70 μL/min was observed at frequency of 125 Hz.
Yamahata
et al. [52] reported a PMMA micropump with electromagnetic actuation. The maximum flow rate of 400 μL/min and back pressure of 1.2 kPa were observed at resonant frequencies of 12 Hz and 200 Hz. Su
et al. [53] reported the analysis and fabrication of a valveless electromagnetic
micropump with two parallel flexible diaphragms. The maximum flow rate of 6 μL/s and the
displacement of 0.30 mm were observed at 100 Hz frequency with 0.3 A induced current.
Balaji
et al. [54] reported the design, fabrication and testing of a flat pump with millimeter thickness. The maximum flow rate of 15 μL/min was observed at applied voltage of 2.5 V with 68 Hz frequency and 19 mA current. Yu-feng
et al. [55] reported a parallel dynamic micropump with valve, diaphragm
and electromagnetic coil. The maximum flow rate of 6 μL/s and the diaphragm displacement of 30 μm
were observed at 100 Hz frequency with 0.3 A of current. Shen
et al. [56] fabricated and characterized a
reciprocating PMMA ball valve micropump with electromagnetic actuation. The micropump showed a
backpressure of 35 kPa and flow rate of 6 mL/min at 2 W electromagnetic actuation power with 20 Hz
resonant frequency. Halhouli
et al. [57] worked on the design of a novel electromagnetic pump that
based on the rotation of two hard magnets kept in channel, with opposing polarity. The maximum flow
rate of 13.7 mL/min at 200 rpm and a pressure of 785 Pa at 136 rpm were observed.
2.2.5. Bimetallic Micropumps
Bimetal refers to an object that is composed of two different metals jointed together. The thermal
expansion coefficients of these metals are different. The deflection of a diaphragm made of bimetallic
materials is induced against thermal alternation as long as the two chosen materials possess adequately
discriminative thermal expansion factors. A block diagram of bimetallic micropump is shown in Figure 7.
Figure 7. Bimetallic micropump.
Zhan
et al. [58] reported Si based bimetallic micropump with 10 μm thick layer of aluminum (Al)
on Si substrate. The flow rate of 45 μL/min and back pressure of 12 kPa were observed at applied
voltage of 5.5 V with 0.5 Hz frequency. Zou
et al. [59] designed a micropump that operated on both
bimetallic thermal actuation and thermal pneumatic actuation mechanisms. When the bimetallic
actuator made of Al/Si membrane was heated, the membrane deformed in downward direction. At the
Int. J. Mol. Sci. 2011,
12
same time, the gas in the air chamber expended due to the heat to support bimetallic actuation. The flow rate of 336 μL/min was achieved when the open pressure was 0.5 kPa. A novel micropump operated on bimetallic and electrostatic actuation mechanisms was reported by Pang
et al. [60].
Experimental results showed that the on/off flow ratio of the micropump was 180. Yang
et al. [61]
presented a bimetallic thermally actuated membrane micropump that consisted of two chips, pump
chamber, two bimetallic actuators and two check valves. The maximum flow rate of 43 μL/min was
achieved at applied voltage of 16 V and 0.9 Hz frequency. The forces generated through bimetallic
actuation are large and the implementation is simple. Usually the thermal expansion coefficients of
materials that are involved in bimetallic micropumps are small. That is why the diminutive deflections
are achieved in bimetallic actuation mechanism. The bimetallic micropumps require low voltage
values as compared to other micropump types. But the drawback of bimetallic micropumps is that they
are not suitable to work at high frequencies.
2.2.6. Ion Conductive Polymer Film (ICPF) Micropumps
ICPF actuator shows high speed response. However, the positioning control is difficult. The core
layer of ICPF is made of a sort of perfluorosulfonic acid polymer. Physically it looks like a ―sandwich‖ diaphragm between two thin films that are placed on both sides of the polymer. These two films have
high electrical conductivity. One end of the diaphragm is fixed and the ICPF diaphragm can be
controlled by bending in the direction of either upside or downside as long as an appropriate pair of
voltages is applied at the electrodes. The ICPF actuator is commonly called an artificial muscle
because of the large bending displacement, low actuation voltage and biocompatibility. A schematic of
ICPF and the bending principle is shown in Figure 8.
Figure 8. ICPF micropump.
ICPF actuators have been developed for various applications. Guo
et al. [62] reported a new model
of micro catheter with active guide wire that had two bending degrees of freedom using ICPF actuator.
Tadokoro
et al. [63] developed multi-degree-of-freedom (DOF) micro motion devices using ICPF soft
Int. J. Mol. Sci. 2011,
12
gel actuator. Guo and Asaka [64] proposed an underwater fish like microrobot using ICPF actuator as
the servo actuator swimming motion with three degrees of freedom. Nguyen
et al. [65] reported the
design and fabrication of a flap valve ionic polymer/metal composite micropump with the diaphragm supported by a flexible material. A maximum flow rate of 760 μL/min and backpressure of 1.5 kPa were observed at the applied voltage of 3 V with 3 Hz frequency. Chen
et al. [66] proposed the design
of an integrated sensory actuator. The polyvinylidene fluoride (PVDF) films were used for
simultaneous feedback of bending and force outputs of the actuator. Fang and Tan [67] proposed a
control oriented model to envisage the deformation of diaphragm and the flow rate. Experimental
results of the polypyrrole (PPy) actuated micropump showed that the maximum flow rate of 1260 μL/min was observed at the voltage of 4 V.
2.2.7. Phase Change Micropumps
The basic principle used in phase change type actuators and micropumps is the vaporization and
condensation phenomenon. In vaporization, the phase transition occurs from liquid phase to vapor
phase. While in condensation, the change of the physical state occurs from gaseous phase to liquid
phase. The phase change type micropump consists of a heater, diaphragm and working fluid chamber.
A schematic of phase change micropump is shown in Figure 9.
Figure 9. Phase change micropump.
Sim
et al. [68] proposed a phase change type micropump consisting of a pair of Al flap valves and a
phase-change type actuator. The actuator comprised of a heater, working fluid chamber and silicone
rubber diaphragm. The diaphragm was actuated by the vaporization and the condensation of the
working fluid in the chamber of the pump. The maximum flow rate of 6.1 μL/min was achieved at
applied voltage of 10 V with 0.5 Hz frequency and 60% duty ratio for zero pressure difference.
Boden
et al. [69] reported a high pressure micropump with polymeric paraffin actuation. The flow rate
of 74 μL/min was achieved at a low voltage waveform with water as a pumping fluid. When the
pressures up to 1MPa were applied on the valves, the micropump showed no leakage. Sim
et al. [70]
reported the fabrication and testing of a micropump comprised of a pair of Al flap valves and a phase
change type actuator. The actuator was composed of a heater, diaphragm and fluid chamber. The
maximum flow rate of 97 μL/min was observed at applied voltage of 8 V with 70% duty ratio and 2 Hz
frequency for zero pressure difference.
Int. J. Mol. Sci. 2011,
12
2.2.8. Shape Memory Alloy (SMA) Micropumps
SMA are the metals which exhibit two very unique properties such asand the
They have the capability of changing their shapes upon application of an external
stimulus. The SM effect involves a phase transformation between two solid phases. At high temperature
the phase is called austenite and at low temperature the phase is called martensite. SMA starts in
martensite phase and transforms into austenite phase after being heated. This property of materials is
useful to make SMA micropumps. A schematic of an SMA micropump is shown in Figure 10.
Figure 10. SMA micropump.
The first thin film SMA micropump with two different actuation configurations was reported by
Benard
et al. [71]. The pump was driven by an electrical drive signal provided directly through the
Titanium/Nickel (Ti/Ni) thin films, resulting in Joule heating induced phase transformation that
initiated the SM effect. The maximum flow rate of 50 μL/min was observed at 0.9 Hz frequency.
Makino
et al. [72] reported the development of SMA actuated micropump to use in micro analysis and
micro dosage systems. The maximum flow rate of 0.4 μL/cycle was observed at a bias pressure of 100 kPa.
Xu
et al. [73] developed a micro SMA pump composed of a NiTi/Si composite membrane, pump
chamber and two inlet/outlet check valves. The flow rate of 340 μL/min and back pressure of 100 kPa
were achieved. Shuxiang and Fukuda [74] developed SMA micropump composed of SMA coil
actuator, two diffusers, pump chamber and a casing. The maximum flow rate of 500–700 μL/min was
achieved by changing the frequency. Zhang and Qiu [75] reported a Ti/Ni/Copper (Cu) shape memory
thin film micropump comprised of a TiNiCu/Si driving membrane, pump chamber and two inlet and outlet check valves. The hysteresis width ∆T of 9 °C was observed. Setiawan [76] reported the performance assessment of SMA spring as actuator for gripping manipulation. The SMA actuator was
a TiNi tensile spring with diameter of 50 mm wire and 350 gram hanging mass. SMA have many
attractive properties like high force to volume ratio, ability to recover large transformation stress and
strain upon heating and cooling processes, high damping capacity, chemical resistance and
biocompatibility. Usually the deformation of SMA cannot be precisely controlled and investigated due
to temperature sensitivity. Additionally, the designs based on TiNi film devices with more practical,
effective and complex characteristics, are required through multiple DOF and compact structures.
Recently reported mechanical micropumps are listed in Table 1.
Int. J. Mol. Sci. 2011,
12
Table 1. Recently reported mechanical micropumps.
Actuation
Materials used
Pressure/
Reference
Frequency
for fabrication
(μL/min)
Pressure
Polycarbonate (PC),
Liu et al.
PMMA, PDMS, PZT,
2010 [33]
Zhu et al.
Polyetheretherketone/
2009 [77]
PDMS/Metal/Ceramics
2011 [78]
Halhouli
et al.
2010 [57]
Shen et al.
2009 [79]
Lee et al.
2009 [39]
Teymoori
2005 [80]
Int. J. Mol. Sci. 2011,
12
Table 1. Cont.
Reference
Chia et al.
2010 [47]
Tan et al.
2010 [48]
Zou et al.
1997 [59]
Stainless steel,
Sim et al.
Silicone rubber,
2008 [70]
Ti, Nickel (Ni),
2006 [75]
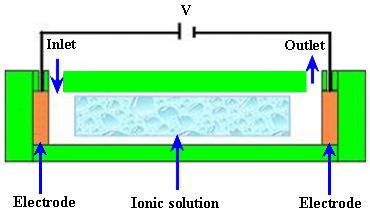
Int. J. Mol. Sci. 2011, 12
2.3. Non-Mechanical Micropumps
The non-mechanical micropumps have no moving mechanical part so that generally they need a
type of mechanism that can convert non-mechanical energy into kinetic momentum. In general, non-
mechanical pumps do not need physical actuation components so the geometry, design and fabrication
of these micropumps are relatively simple and easy. These micropumps have certain limitations, such
as the use of only low conductivity fluids and the actuation mechanisms interfere with the pumping
liquids. A detailed description of non-mechanical micropumps is given below.
2.3.1. Electroosmotic (EO) Micropumps
EO flow is the motion of the liquid that is induced by an applied potential across a capillary tube or
microchannels. The fluid with electric conductivity feature is driven by appropriately exerting an
external electrical field upon the channel walls that are naturally charged. A schematic diagram of an
electroosmotic micropump is shown in Figure 11.
Figure 11. EO micropump.
Zeng et al. [81] fabricated an EO micropump that used DI water as working fluid. The maximum
flow rate of 3.6 μL/min and pressure of 2026.5 kPa were obtained at applied voltage of 2 kV. Takemori et al. [82] reported an EO micropump with high pressure. The flow rate of 0.47 μL/min and
pressure of 72 kPa were observed at applied 3 kV. Hu and Chao [83] investigated the EO flow in EO
micropump with an overlapped electrical double layer (EDL). The results showed that the flow was
relatively different from the channel with a dimension greater than the EDL, which demonstrated plug
like flow properties. Good et al. [84] performed the mathematical modeling and experimental testing
of water activated micropump that was actuated using the osmotic effect. The maximum flow rate of 17 μL/min/mg of dry polymer particles, with a 355–425 μm diameter, was achieved. Ryu et al. [85] proposed a biodegradable osmotic micropump for long use and controlled discharge of basic fibroblast
growth factor (bFGF). The release of bFGF was regulated at a rate of 40 ng/day for duration of four
weeks. Yairi and Richter [86] developed an EO micropump based on voltage control. The flow rate of
0.054 mL/min and pressure of 5.5 kPa were achieved. Borowsky et al. [87] fabricated a high pressure
EO micropump and tested the performance of fluid dynamic. The maximum flow rate of 85 μL/min
and pressure of 25 atm were achieved. Wang et al. [88] reported the general characteristics, fabrication
technologies and applications of EO micropumps. The transport of various solutions compositions into
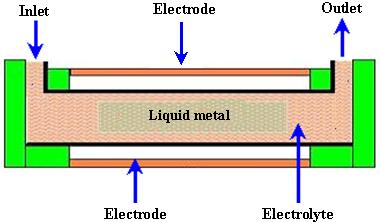
Int. J. Mol. Sci. 2011, 12
capillaries can cause problems in the flow constancy of an EO pumped system in some applications.
Sometimes flow rates are modified due to adsorption of compounds from the samples or sample matrix
on the surfaces of the pumping elements. This problem can be solved by separating the pump fluid
from the sample and reagent solutions in the analytical system.
2.3.2. Electrowetting (EW) Micropumps
EW is a microfluidic phenomenon that is currently used as a driving mechanism for fluidic devices.
EW involves modifying the natural surface tension or capillary forces intrinsic to an oil and water
interface at small length scales. At less than 1 mm distance, the electrical and surface tension forces are
much stronger than gravity. The digital EW is applied to control the surface tension between solid
phase electrode and liquid phase droplet. A schematic of an EW micropump is shown in Figure 12.
Figure 12. EW micropump.
Yun et al. [89] reported a continuous EW micropump. For the actuation energy of micropump, the
surface tension induced motion of mercury drop in a microchannel filled with electrolyte was used. The micropump consisted of a stack of three wafers bonded together. The flow rate of 70 μL/min and pressure of 0.8 kPa were achieved at 2.3 V with frequency of 25 Hz and power consumption of 170 μW. Hoshino et al. [90] reported the pico liter liquid actuation in a microinjector by using a pulled glass tube as the device structure. The tube caused pumping and ejection by EW on dielectrics.
500 picoliter water was pumped up at the maximum applied voltage of 1400 V. In pumping pressure,
an increase value of 0.6 Pa was calculated. Colgate and Matosumoto [91] reported a detailed model of
a test device showing liquid flow in a small channel for the study of EW. EW gives direct fluid
pumping without any moving mechanical parts that can be valuable in many application areas of
microelectronic devices. The initial results showed that EW might be used to get pressures on the order of 0.01 MPa in a 10 μm radius channel. Chang et al. [92] reported the driving characteristics of the EW-on-dielectric device with aluminum oxide (Al2O3) deposited by using the method of atomic layer deposition. When the voltage was applied between control electrode and reference electrode then the
flow of 2 μL for water droplet in an air environment was achieved.
2.3.3. Electrochemical Micropumps
The most common feature of electrochemical micropumps is the generation of bubbles by
electrolysis in which the decomposition of water occurs into its constituents, such as hydrogen gas
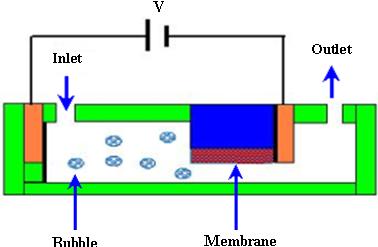
Int. J. Mol. Sci. 2011, 12
(H2) and oxygen gas (O2), when the current is passed through water. During this mechanism, the key
component is a bubble reservoir filled with a redox electrolyte solution. The reaction of electrolysis
can be described by the equations (9) and (10).
A schematic of electrochemical micropump is shown in Figure 13.
Figure 13. Electrochemical micropump.
Suzuki and Yoneyama [93,94] fabricated an electrochemical syringe pump by using micromachining
for low operating voltage and power consumption. A microfluidic system was developed by integrating
an on-chip micropump and check valves that worked through a H2 bubble generated electrochemically. Thin film electrodes were used with a platinum black working electrode. PDMS substrate was used to
make flow channels and containers for electrolyte solutions. Two dye solutions were transported and
merged in a flow channel and sheath flows were observed. Yoshimi et al. [95] developed an artificial
synapse using the electrochemical micropump. The micropump consisted of a glass nozzle and two
blackened platinum electrodes filled with a neurotransmitter solution for the electrolysis process. To
drive the solution towards the neuron, a potential difference of 3.0 V was applied to the electrodes.
Kim et al. [96] reported a PPy-membrane microfluidic pump. The pumping action was stimulated by an
electrochemical actuated PPy-PDMS membrane. The check valves were used to control the direction of
flow. The maximum flow rate of 52 μL/min was obtained at ±1.5 V with input power of 55 mW.
2.3.4. Evaporation Micropumps
In evaporation micropumps, a controlled evaporation of liquid is used. Evaporation is a process in
which liquid is converted from its liquid form to vapor form. The reverse of this process is known as
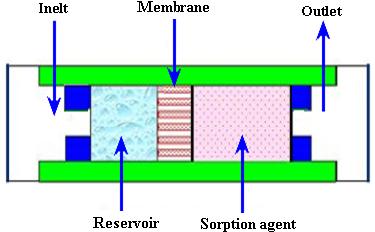
Int. J. Mol. Sci. 2011, 12
condensation. The pumping principle of the evaporation type micropump is the same as the xylem
transport system in plants. A schematic of an evaporation micropump is shown in Figure 14.
Figure 14. Evaporation micropump.
Effenhauser et al. [97] reported the evaporation based disposable micropump for continuous
monitoring systems. The controlled evaporation of liquid was done through a membrane into gas space
that contained a sorption agent. In the gas chamber, the vapor pressure was kept lower than saturation.
During this process, the fluid evaporation from membrane was substituted by capillary forces that
resulted in a flow from the reservoir. The average flow rate of 0.35 μL/min was achieved.
Namasivayam et al. [98] reported the micropump based on the generally observed phenomenon of
transpiration in plant leaves for continuous very low flow rates. As the vapor diffused out due to
heating, a new transport of liquid was supplied into the channel from a reservoir for steady state
operation. Guan et al. [99] reported a micropump based on capillary-evaporation effects for a microfluidic flow injection chemiluminescence system. The average flow rate of 3.02 μL/min was achieved with an ambient temperature of 20–21 °C and relative humidity of 30–32% for fluctuation
within 2 h. Heuck et al. [100] reported the evaporation-based micropump integrated into a scanning
force microscope probe for the flow of liquid through its hollow cantilever and tip areas. A flow rate of
11 pL/s was obtained at room temperature.
2.3.5. Bubble Micropumps
The bubbles micropump is based on periodic expansion and collapse in the volume controlled by
voltage input. The volume change in chamber is incorporated with the diffuser/nozzle mechanism that
is used to determine the direction of fluidic flow. The bubbles are generated by heating process. A
schematic of the bubble micropump is shown in Figure 15.
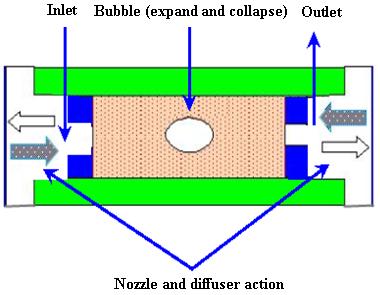
Int. J. Mol. Sci. 2011, 12
Figure 15. Bubble micropump.
Tsai and Lin [101,102] reported a valveless thermal-bubble micropump. Later they developed a
microfluidic mixer system with a gas bubble filter using the bubble micropump. The maximum flow rate of 5 μL/min was achieved at 250 Hz with applied periodic voltage, 10% duty cycle and power consumption of 1 W. Lew et al. [103] developed a collapsing bubble micropump. The bubbles with a
radius of about 3–5 mm were investigated through the experimental set up that employed a low voltage
electrical spark of 55 V created with a capacitor for bubble generation. It was reported that the
proposed theory could also work with even smaller bubbles. Jung and Kwak [104] reported the
fabrication and testing of bubble type micropumps using an embedded microheater. The micropump
comprised of a pair of nozzle/diffuser, flow controller, microchannels and a pumping chamber. The
maximum flow rates of 6 μL/min at duty ratio of 60% for circular chamber and 8 μL/min at duty ratio
of 40% for the square chamber were achieved. Cheng and Liu [105] reported an electrolysis-bubble
micropump based on the roughness-gradient design in the microchannel. The electrolysis actuation and
the surface tension effect were used for the micropump. The maximum flow rate of 114 μL/min was
obtained at applied voltage of 15 V with a frequency of 4.5 Hz. Chan et al. [106] developed a bubble
type micropump with high frequency flow reversal using embedded electrodes in a closed microfluidic
microchannel. The micropump consisted of a microfluidic chamber and microelectrodes on a glass
substrate that was assembled by PDMS-sheet. The maximum flow rate of 37.8 μL/min was achieved at
2.3.6. Magnetohydrodynamic (MHD) Micropumps
MHD is a field in which the dynamics of electrically conducting fluids is studied. The Lorentz force
is the driving source perpendicular to the electric and magnetic fields for MHD type of micropumps.
The working fluid is selected to achieve conductivity of 1 s/m or higher, in addition to externally
providing electric and magnetic fields. The Lorentz force can be expressed by the equation (11).
Where, is force, is electric field, is instantaneous velocity of particles, is magnetic field and
is electric charge of the particle.
A schematic of the MHD micropump is shown in Figure 16.
Int. J. Mol. Sci. 2011, 12
Figure 16. MHD micropump.
Jang and Lee [107] reported the MHD micropump. The pressure head difference of 18 mm at
38 mA and a flow rate of 63 μL/min at 1.8 mA were achieved with an inside diameter of 2 mm for
inlet/outlet tube and a magnetic flux density of 0.44 T. Zhong et al. [108] reported the fabrication of
MHD micropump using ceramic tapes. Experiments were performed using mercury slugs, saline
solutions and DI water. Eijkel et al. [109] developed a circular ac MHD micropump for
chromatographic applications. The device comprised of a glass-gold-laminate-glass sandwich structure
with the channel defined in the electroformed gold layer. Reversible flow rate of 40 μm/s was
achieved. Patel and Kassegne [110] reported a MHD micropump with EO-thermal effects using
3D-MHD equations. The use of a developed numerical framework, flow channel geometries, Joule
heating, effects of non-uniform magnetic/electric fields and EO in MHD micropumps were
investigated. Duwairi and Abdullah [111] developed a model to envisage the fluid flow in the MHD
micropump. By applying the finite difference method and the SIMPLE algorithm, the transient,
incompressible, laminar and flow equations were numerically solved. Kang and Choi [112] reported
the design and fabrication of MHD micropump with a mixing function in which the fluids were mixed
and pumped at the same time by coupling between Lorentz force and the moving force of an electric
charge in the electric field.
2.3.7. Flexural Planer Wave (FPW) Micropumps
The FPW micropumps are driven ultrasonically. The fluidic motion induced by traveling FPW can
be used for the transport of liquids. The liquid motion is in the direction of wave propagation and the
speed is proportional to the square of acoustic amplitude. Low operating voltage is required for
acoustic streaming. A schematic of the FPW micropump is shown in Figure 17.
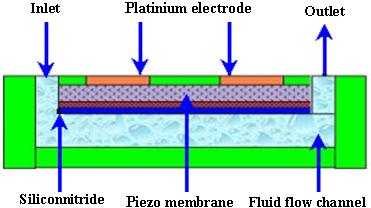
Int. J. Mol. Sci. 2011, 12
Figure 17. FPW micropump.
Moroney et al. [113] reported the process of water pumping induced by 4.7 MHz ultrasonic Lamb
waves. The waves were moving in a composite membrane of silicon nitride and piezoelectric zinc
oxide with a thickness of 4 μm. The observed speed was 100 μm/s at the applied rf voltage of 8 V with
6.5 nm wave amplitude. Nguyen and White [114] reported the design and numerical model of an
ultrasonic FPW micropump and microfluidic system. The effects of channel height, wave amplitude,
and backpressure on the velocity and flow rate were studied. The influence of thermal transport of the
acoustic streaming was also investigated. Results showed that the micropumps with channel heights of
a few micrometers exhibited high-quality performance because the flow rate and hydraulic impedance
against backpressure were high. Nguyen et al. [115] reported a FPW micropump integrated with flow
sensor for in situ measurement. The FPW micropump and the flow sensor made a complex
microfluidic system capable of controlling the fluid flow in the device. Meng et al. [116] reported the
ultrasonic FPW micropump. The waves travelled along a thin membrane to stimulate an acoustic field
in the fluid that was in contact with the membrane. The micropump with a combination of radial
transducers and unidirectional fluid flow resulted in a flow speed of 1.15 mm/s. Jang et al. [117]
investigated the actuating frequency control of acoustic-streaming flow patterns in a diaphragm driven
microfluidic chamber. Microfluidic circulatory flow was achieved using the resonant vibration of
diaphragms. Experiments were performed to study in-plane velocity profiles near the interface of
circulations where the acoustic intensity was measured to be large. The proposed flow process was
reported to be useful for pumping, active mixing and particle focusing applications. Singh and
Bhethanabotla [118] studied the enhancement in the efficiency of acoustic-streaming. Microfluidic and
biosensing applications of surface-acoustic wave devices depend on the acoustic-streaming process
resulting from high intensity sound waves that interact with the fluid medium.
2.3.8. Electrohydrodynamic (EHD) Micropumps
In an EHD micropump, the force is generated by the interaction of electric field and mobile charges
in the fluid. These pumps have emitter and collector electrodes that are regularly spaced along a
microchannel and require no moving parts such as impellers, bellows or valves. The electrical charges
generated from the electrodes mobilize according to the direction of the electric field that is built up by
Int. J. Mol. Sci. 2011, 12
the electrodes and tract in the surrounding liquid molecules to move together by the ion dragging force.
The force acting on the fluid is given by the equation (12).
Where, is force on fluid, is current, is distance between electrodes, is ion mobility coefficient of
the dielectric fluid. A schematic of an EHD micropump is shown in Figure 18.
Figure 18. EHD micropump.
Ritcher and Sandmaier [119] fabricated the first dc charged injection EHD micropump comprised of
two electrically isolated grids. The flow rate of 15,000 μL/min and the pressure head of 1.72 kPa were achieved at applied voltage of 800 V. Fuhr et al. [120] developed the first EHD micropump based on
travelling wave-induced electroconvection. The flow rates of 0.05–5 μL/min were achieved.
Darabi et al. [121,122] reported the EHD polarization micropump for electronic cooling and EHD ion
drag pump. The model devices exhibited a maximum cooling capacity of 65 W/cm2 with pumping
head of 250 Pa. Yang et al. [123] reported an ejection type EHD micropump using indium-tin-oxide
(ITO) planar electrodes to deal with the aging problem. The planar electrodes could drive the ethyl
alcohol with a flow rate of 356 μL/min at applied dc voltage of 61 V. Lin and Jang [124] reported the
numerical microcooling analysis for EHD micropump. The micropump offered the pumping power
using the dipole moment force generated from polarizing fluid molecules. The pressure head of
13 kPa and wall heat flux of 10 W/cm2 were observed at applied voltage of 500 V with pitch of
500 μm for parallel electrodes. Darabi and Rhodes [125] reported the computational fluid model of ion
drag EHD micropump. The micropump consisted of an array of interdigitated electrodes with the top
and bottom parts of the channel. Singhal and Garimella [126] reported induction based EHD
micropump for high heat flux cooling process. The numerical model was developed by solving the three
dimensional transient fluid flow and charge transport problem due to simultaneous actuation of EHD and
the vibrating diaphragm. Recently reported non-mechanical micropumps are listed in Table 2.
Int. J. Mol. Sci. 2011, 12
Table 2. Recently reported non-mechanical micropumps.
Material used
Actuation
Pressure/
Reference
Frequency
Fabrication
(μL/min)
Pressure
Chan et al.
2010[106]
2007 [104]
et al.
2009 [127]
Singhal and
Garimella
2007 [126]
Lister et al.
2010 [128]
buffer, DI water
Xu et al.
2010 [129]
Choi 2010
Int. J. Mol. Sci. 2011, 12
Table 2. Cont.
Reference
Applied Pressure
Lim and Choi
Si, Pyrex glass,
2009 [130]
Yun et al.
2002 [89]
Kim et al.
2008 [96]
Heuck et al.
2008 [100]
Guan et al.
2006 [99]
Luginbuhl
1997 [131]
Int. J. Mol. Sci. 2011, 12
3. Microneedles
Microneedles are very useful delivery devices. These devices provide an interface between the drug
reservoir and the patient's body for releasing or extracting the fluid. The length of microneedles should be long enough that it penetrates the epidermis and short enough not to reach the dermis, in order to
avoid pain. The concept of microneedles was proposed in the 1970s but it was not realized
experimentally until the 1990s when the industry of microelectronics provided the microfabrication
tools essential to make such small structures. The first microneedle arrays reported in the literature
were developed by etching the Si wafer for intracellular delivery [132]. These needles were inserted
into cells and nematodes to increase molecular uptake and gene transfection. After that a number of
attempts have been made by various researchers to develop the fabrication processes and different
designs of microneedles. MEMS technology is the most promising to fabricate the optimal design of
microneedles for particular applications. The typical diameter and length of MEMS-based
microneedles are in the range of micrometers. These microneedles are different from standard
hypodermic needles used in biomedicine. Generally, the length of the MEMS-based microneedles is
less than 1 mm. Thus microneedles are significantly smaller in length than ordinary needles [4,133].
Microneedles or microneedle arrays can be used as a stand-alone microfluidic device as well as part of
biological detection, fluid extraction or delivery system. Microneedles can be integrated with
micropumps, biosensors, microelectronic devices and microfluidic chips.
3.1. Categories of Microneedles
Different designs of microneedles have been reported in literature for various applications.
Microneedles can be classified in various ways such as according to structure, overall shape, tip shape,
length, array density, material used for fabrication and applications [3,4]. Details of microneedle
categories are shown in Table 3.
Table 3. Categories of microneedles.
Structure
Tip Shape
Material Used
Application
Single crystal silicon Drug delivery
Blood extraction
Out-of-plane Candle
Microhypodermis PGA
Allergies diagnosis
Animal identification
Ink-jet printing
Sensing electrodes
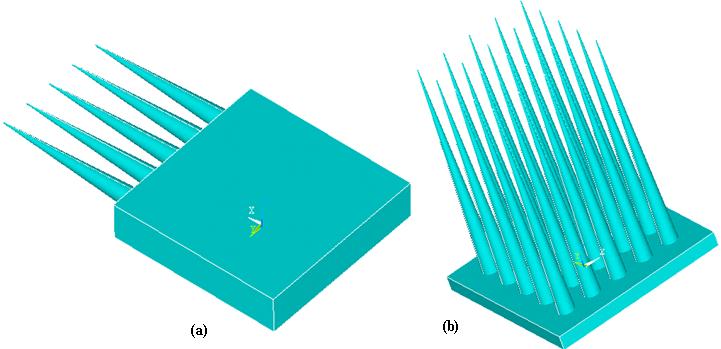
Int. J. Mol. Sci. 2011, 12
3.1.1. Structure of Microneedles
Structure is the most important consideration for microneedles design and fabrication. Based on the
fabrication process, the microneedles are classified in two types.
In-plane microneedles Out-of-plane microneedles
In in-plane microneedles, the microneedle shafts or lumens are parallel to the substrate surface. The
major advantage of in-plane microneedles is that the length of the microneedles can be easily and
accurately controlled during fabrication process. The limitation of in-plane microneedles is that it is
very difficult to fabricate microneedle arrays with 2D geometry. In out-of-plane microneedles, the
lengths of the microneedles protrude out of the substrate surface and it is easier to fabricate
out-of-plane microneedles in 1D or 2D arrays. However, fabrication of out-of-plane microneedles with
length and high aspect ratio structure is challenging [4,134]. A schematic illustration of in-plane and
out-of-plane microneedles is shown in Figure 19.
Figure 19. (a) In-plane microneedles; (b) Out-of-plane microneedles.
In-plane microneedles were developed in the 1980s [134] and not intended for drug delivery or
fluid transport. An implantable ten-channel microelectrode recording array with an on-chip signal
processing probe was fabricated for long term recording of neural bio-potentials. The length of probe
and thickness were 4.7 mm and 15 μm respectively. A 1D array of micro neural probes [135] and more
sophisticated 2D array have been developed [136]. After that various attempts have been made to
develop in-plane microneedles for different applications. The major drawback associated with in-plane
microneedles is the limited density. To overcome this limitation, out-of-plane microneedles have been
developed. One of the earliest out-of-plane microneedle array consisted of 100 microneedles with a
length of 1.5 mm was reported in 1991 [132].
Microneedles can also be categorized as solid or hollow according to the structure. Hollow needles
were invented in 1844 [137] and gained increasing importance in the biomedical field. There are no
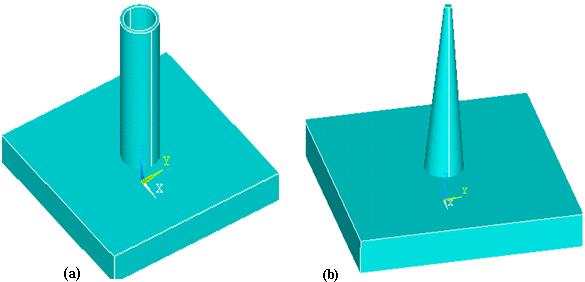
Int. J. Mol. Sci. 2011, 12
other effective ways to transport the fluid into the human body [138]. Hollow needles have become
more important after the invention of microneedles. Hollow microneedles have an internal bore or
lumen which allows flow of fluid/drug through the microneedles. A combination of surface and bulk
micromachining techniques was used to fabricate hollow in-plane microneedles with 1-6 mm length
and fully enclosed channels of silicon nitride [139]. The channels were 9 μm in height. The solid
microneedles have solid lumens and exhibit more strength than hollow microneedles. Solid
microneedles can be further categorized into coated and dissolving microneedles. In coated
microneedles, the drug particles are coated on lumen surface and injected into patient body. The
microneedles are withdrawn from the body after dissolution of the coated drug. In dissolving
microneedles, the base is non-dissolving and withdrawn from the skin after dissolution of the
microneedles. Various types of solid coated and dissolving microneedles have been reported [19].
Coated Ti microneedles arrays with a length of 190 μm have been reported for the delivery of
parathyroid hormone (I—34) in human body for the treatment of osteoporosis by Zosano Pharma, Inc.
(formerly Macroflux®, ALZA Corp.) [140]. The successful delivery of drug depends on the methods
used for coating of microneedles [141,142]. References [143,144] fabricated the first out-of-plane
sharp solid microneedles for drug and gene delivery. A schematic of hollow and solid microneedles is
shown in Figure 20.
Figure 20. (a) Hollow microneedle; (b) Solid microneedle.
Hollow silicon dioxide (SiO2) microneedles have been fabricated using deep reactive ion etching
technique [145]. Reference [146] fabricated SiO2 microneedles which mimic a jagged mosquito's needle. In-plane hollow metallic hypodermic microneedles and microneedle array were reported using
electroplated palladium (Pd) alloys and Ni [147–149]. Using a combination of isotropic and an
isotropic etching process, sharp tip hollow out-of-plane single crystal Si microneedles were
fabricated [150]. One of the earliest solid microneedles design was in the form of pyramidal Si
microprobes [151]. Sharp Si solid microstructures with a height of 150 μm were fabricated with
anisotropic dry etching technique using SF6 and O2. Such type of solid microneedles was used to increase permeability of human skin up to fourth order of magnitude in vitro. Solid microneedles for
TDD were reported for the first time in 1998 [144].
Int. J. Mol. Sci. 2011, 12
3.1.2. Shape of Microneedles
The shape of the microneedle is very critical and important during design and fabrication.
Microneedles can be classified on the basis of overall shape and tip shape. Different designs of
microneedles have been proposed and fabricated such as cylindrical, canonical, pyramid, candle, spike,
spear, square, pentagonal, hexagonal, octagonal and rocket shape [3,4]. Microneedles have also been
reported with various tip shapes like volcano, snake fang, cylindrical, canonical, micro-hypodermis
and tapered. Schematic illustrations of various designs of microneedles with respect to shape and tips
are shown in Figure 21.
Figure 21. Shapes of microneedles (a) Cylindrical; (b) Tapered tip; (c) Canonical;
(d) Square base; (e) Pentagonal-base canonical tip; (f) Side-open single lumen; (g) Double
lumen; (h) Side-open double lumen.
Rocket shape microneedles have been fabricated using two photon polymerization method [152].
Octagonal solid out-of-plane Si microneedle array has been fabricated for drug delivery [153]. Solid
Si-tip microneedles have been fabricated using wet etching technology [154]. Pyramidal out-of-plane
Si microneedle array has been fabricated by wet etching for transcutaneous drug delivery [155]. Side
opened sharp tip out-of-plane solid microneedle has been fabricated by hot embossing to improve skin
permeability for hydrophilic molecules [156]. Cylindrical hollow out-of-plane microneedles with
tapered tip using combination of ICP etching have been fabricated for TDD [4].
Int. J. Mol. Sci. 2011, 12
3.1.3. Materials Used for Microneedles
Microneedles can be classified on the basis of materials. Material selection is very important to design
and fabricate microneedles for any particular application. Many researchers used Si for microneedles
fabrication [4,157–162], which is a brittle material [163] and can be harmful to health. Different
researchers have understood this critical issue and used polymeric material instead. Most polymers have
a strong history of biocompatibility. They exhibit excellent mechanical and chemical properties [164]
that are suitable for microneedle fabrication. Fabrication of microneedles has been reported using various
polymers such as (Polyglycolic acid) PGA, (Poly-L-Lactide acid) PLLA, PC, PDMS, PMMA, etc.
Fabrication of polymeric microneedles has been reported by various researchers [165,166]. Some other
materials have also been reported such as glass, metal, alloy, etc. [4]. Glass hollow elliptical tip
microneedles have been fabricated using micropipette pulling technique for intrascleral delivery [167].
In-plane Ti microneedles have been fabricated using bulk micromachining for drug delivery [168].
Tungsten microneedles have been reported for nerve penetration [169].
3.1.4. Microneedles Applications
On the basis of applications, microneedles can be categorized into various types because different
types of microneedles are suitable for specific applications. The suitable length of microneedles for drug delivery is 100 μm to 300 μm, but for blood extraction the appropriate length of microneedles is 1100 μm to 1600 μm [170]. Solid microneedles are suitable for cell surgery. Microneedles have been reported for drug delivery, blood extraction, fluid sampling, cancer therapy, microdialysis, ink-jet
printing and sensing electrodes. Hollow Ti microneedles have been fabricated for blood extraction
using sputtering and deposition methods [171]. SiO2 hollow square microneedles have been reported for flow delivery systems using electrochemical etching technique. Hollow out-of-plane SiO2 microneedles have been fabricated using lithography for cell surgery [162]. Stainless-steel hollow and
solid microneedles have been reported using surface micromachining and etching techniques for
dermal diphtheria and influenza vaccination [172]. Hollow out-of-plane Si microneedles have been
fabricated for TDD [4]. The extensive detail of materials used for microneedle's designs, structure,
array size, fabrication techniques, analysis and application has been presented in Table 4, Table 5
Int. J. Mol. Sci. 2011, 12
Table 4. Recent review of silicon microneedles.
Structure of
Shapes of
Fabrication
Reference
Material
Dimensions
Analysis type
Application
techniques
Waseem et al.
Transdermal drug delivery
(Static/Transient)
Chen et al.
Deep reactive ion
Fluidic analysis
Transdermal drug delivery
Zhang et al.
PLGA nano Particles
Transdermal drug delivery
Photolithography
Waseem et al.
Structural/Fluidic
Transdermal drug delivery
(Static/Transient)
Zhang et al.
Drug delivery/fluid
Fluidic analysis
Bi-mask technique
Ding et al.
Fluidic analysis /
diphtheria/influenza
Haq et al.
Transcutaneous drug
Fluidic analysis
Yu et al. 2009
Structural analysis
recording system
Int. J. Mol. Sci. 2011, 12
Table 4. Cont.
Structure of
Shapes of
Fabrication
Reference
Material
Dimensions
Analysis type
Application
techniques
Coulman et
Transdermal/Intradermal
shape/Pointed/Frustum
Diffusion of nano particles
Chen et al.
Transdermal drug
Macro porous tip
Fluidic analysis
Roxhed et
Transdermal drug
Fluidic analysis
Bhandari et
Square base canonical
Donnelly et
Fluidic analysis/Statistical
skin/Porcine skin
Photodynamic therapy
Lee et al.
Conical/Pyramidal
Structural analysis
Notations: L = Length of needle, Wb = Base width, Do = Outer diameter, Di = Inner diameter, Db = Base diameter, Dt = Tip diameter, DP = Depth.
Int. J. Mol. Sci. 2011, 12
Table 5. Recent review of polymeric microneedles.
Structure of
Shapes of
Array size/
Reference
Materials
Dimensions
Analysis type
Fabrication techniques
Application
Park et al.
Diffusion of trypan
Transdermal drug
Canonical/Square base
Gomaa et al.
Permeability with
Laser micromachining
microneedle density
Donnelly et al.
Intradermal delivery
Bodhale et al.
Hot embossing/UV excimer
Side opened/Sharp tip
Structural/ Fluidic
Matteucci et
Rounded tip/Sharp tip
Bevel angle = 30°
al. 2009 [185]
Han et al.
Lithography/Ni electroplating/ PDMS
Grooves-embedded
replication/Hot embossing
Jin et al. 2009
Transdermal drug
L = 200–1500 mm
Drug transportation
DXRL/Hot embossing
Oh et al. 2008
Sharp tip/ Spear
L = 200–500 µm
Molding/Hot embossing
permeability for
hydrophilic molecules
Emam et al.
Int. J. Mol. Sci. 2011, 12
Table 5. Cont.
Structure of
Shapes of
Array size/
Reference
Materials
Dimensions
Analysis type
Fabrication techniques
Application
Wb = 90, 120, 150,
Aoyagi et al.
Straight/Harpoon
Artificial skin of silicone
Etching/Injection
Tip angle = 10°,
20°, 30°, 40°
Hsu et al.
Molding/KOH etching
Notations: L = Length of needle, T = Thickness, Wb = Base width, Do = Outer diameter, Di = Inner diameter, Db = Base diameter.
Table 6. Recent review of SiO2, glass, stainless-steel, and metallic microneedles.
Structure of
Shapes of
Array size/
Fabrication
Reference
Materials
Dimensions
Analysis Type
Techniques
Kim et al. 2010
Vaccine delivery
Kato et al.
Cellular function
Structural (Panitration)
Ding et al.
L = 245, 300–900 µm
Tangentially cut tip
Jiang et al.
pulling technique
Int. J. Mol. Sci. 2011, 12
Table 6. Cont.
Structure of
Shapes of
Fabrication
Reference
Materials
Dimensions
Analysis Type
Techniques
Jin et al.
Transdermal drug
L= 200–1500 mm
Drug transportation
Hou et al.
Transdermal drug
Fluidic analysis
Tetrahedron/Sharp
Transdermal drug
Drug transportation
L = 245, 300 µm
Verbaan et al.
D = 200, 300 µm
Transdermal drug
Beveled angle = 45°
Parker et al.
Pressure Testing
Spare/ Sharp tip
Structural analysis
Tip taper angle = 60°
Shibata et al.
Structural analysis
Fluidic analysis
Tapering angle < 5°
Tsuchiya et al.
Fluidic analysis
Sputter deposition
Blood extraction
Notations: L = Length of needle, T = Thickness, Wb = Base width, Do = Outer diameter, Di = Inner diameter, Db = Base diameter, Dt = Tip diameter.
Int. J. Mol. Sci. 2011, 12
3.2. Forces Experienced by Microneedles during Penetration
Fluid is transported through hollow microneedles while solid microneedles are coated with
pharmaceutical materials to transfer the drugs into patient body. Microneedles are under the influence
of various forces during penetration such as bending, buckling, lateral, axial and resistive. To bear all
these forces, the design of microneedles is very important. Microneedles can break during penetration
into the skin because of these forces. An axial force is more dominant on the tip of microneedle during
insertion. This axial force is compressive and leads to buckling of the microneedle. The microneedles
also experience resistive force exerted by skin. Hence, in order to pierce the microneedle into skin, the
applied axial force must be greater than skin resistance. Due to uneven skin surface or human error
during needle penetration, bending may occur. So, it is very important to study the relation between
microneedle geometry and mechanical properties of the material for accurate microneedle design and
prediction of microneedles failure. The buckling force acting on the hollow microneedle during skin
insertion is given by [4,133,196,197].
Where, is Young's modulus of material, is moment of inertia of cylindrical section and
is length of the microneedle.
Moment of inertia (I) for hollow cylindrical section of microneedle is calculated by equation (14).
Where, is outer diameter and is inner diameter of hollow cylindrical section.
The bending force, which the microneedle can withstand without breaking is given by:
Where, is the distance from vertical axis to the outer edge of the section [3,4,198].
The axial force (compressive force), which a microneedle can withstand without breaking is
Where, is the yield strength of the material and is cross-sectional area of the microneedle tip.
Microneedle experiences 3.18 MPa resistive forces exerted by the skin against penetration of
microneedle. To penetrate the microneedles into skin, the external applied force or pressure should be
greater than the resistive skin force. The resistive force offered by the skin before puncturing is given
by the following equation:
Where, is the required pressure to pierce the microneedle into skin.
As the microneedle penetrates the skin, the resistive force falls drastically [199]. After the skin is
pierced by the microneedle, the only force that acts on the microneedles is the frictional force due to
contact of tissue with the microneedle.
Int. J. Mol. Sci. 2011, 12
3.3. Fabrication of Microneedles
Various fabrication techniques have been developed and used for microneedles fabrication such as
hot embossing [156], photolithography [162], micropipette pulling technique [167], surface
micromachining [172], bi-mask technique [175], laser micromachining [179], micro-molding [181],
deep x-ray lithography [200], DRIE [176], lithography, electroplating, molding (LIGA) [201], UV
excimer laser [202], coherent porous Si etching (CPS) [203], injection molding [204] and ICP
etching [3,4]. In these processes, Si and polymer can be used as substrate materials for
microfabrication. Each fabrication technique has its own advantages and limitations. A detailed
discussion on microneedles fabrication techniques will be presented in a subsequent paper.
Lithography and DRIE techniques are mostly used for fabrication of silicon microneedles.
Deposition and etching are the most important phenomenon during the development of microneedles.
Deep holes or free standing structures can be fabricated in silicon wafer with the help of anisotropic
etching process. These high aspect ratio structures are of considerable interest in developing micro
devices for various applications. The general steps of silicon microneedles fabrication are wafer
cleaning, photoresist coating, soft back, masking, exposure, development, hard back, and lift off. These
steps can be repeated according to requirements with desired parameters. For polymeric microneedles
molding, hot embossing, and laser drilling are promising fabrication techniques. The general steps for
polymeric microneedles fabrication are sheet preparation, mold preparation, heating and pressing
mold, de-molding and laser drilling for different lumens/reservoir.
3.4. Microneedles Testing
The concept of microneedles was introduced three decade ago but the first microneedle array for
TDD was fabricated in 1998. After that, various researchers have been involved in developing the most
suitable fabrication method and optimal design of microneedles for biomedical applications. After
2005, the interests of researchers changed and they shifted their attention towards the testing of
microneedles along with design and fabrication. Most of the research groups have been involved in the
structural analysis and skin penetration tests of microneedles in 2010. The testing of microneedles has
been reported on potato skin, chicken skin [175], mouse skin [156,172,180,187,193], cadaver
skin [167,182], pig skin [173,177], chicken leg, beef liver [204], and human skin [156,174,178,183].
Microneedle patches coated with solid state influenza vaccine have been reported to improve the
effectiveness of the vaccine when tested on mouse skin [190]. Dry coated microinjection arrays have
been developed to deliver HSV-2-gD2 DNA vaccine to sensitive regions of mouse skin [205]. The pretreatment of skin by microneedles was combined with the use of highly water soluble pegylated
naltrexone for its transdermal delivery at different concentrations [206]. A new design of probe
integrated with hollow microneedles for atomic force microscope (AFM) has been developed to realize
cellular function analysis in a single living cell [176]. The geometry of microneedles affects its
strength. The shear strength of hollow silicon microneedles can be increased by variation in
microneedles geometry [207]. Using novel microneedle technology, hydrophobic dye called Nile red
has been delivered into porcine skin [169]. The effect of microneedle geometry has been studied on the
transport of a fluorescent dye into human skin [208]. To envisage the effect of microneedle geometry
Int. J. Mol. Sci. 2011, 12
and force of application, optical coherence tomography has been used by penetrating microneedles
arrays into neonatal porcine skin [209]. Short densely packed microinjection array has been developed
to see the effect of strain rate on the precision of penetration into human skin [210]. Reference [211]
has investigated that influenza virus like particles coated on microneedles can cause stimulatory effect
on langerhans cells in human skin. The super short microneedles have been fabricated using Si wet
etching technology and tested for TDD into human skin [212]. The separable arrowhead microneedles
have been introduced and tested for painless delivery of drugs and vaccines into human cadaver
skin [213]. A minimally invasive system has been developed using microneedle electrode array to
deliver macromolecular drugs to the deep skin tissues and tested on hairless rat skin [214]. Solid
silicon microneedles arrays have been used with different lengths and geometry to penetrate epidermal
membrane of human cadaver skin [215]. The microneedles coated with influenza virus like particles
have been used to test the immunogenicity and protective efficacy after vaccination of mice skin [216].
Microneedles rollers have been developed and tested on human and porcine skin to increase skin
permeability and to treat skin for cosmetic purposes [167]. Microneedles have been used to deliver
PLGA (poly-lactic-co-glycolic-acid) nanoparticles in the human skin [174]. Solid polymeric
microneedles have been developed to investigate the transepidermal water loss measurements of
dermatomed human skin [183]. The efficacy of transdermal delivery of insulin has been investigated
by using microneedles rollers on diabetic rats [217]. The administration of virus like particles influenza
vaccine has been studied using microneedles patch on lungs and bone marrow cells of mouse [218].
Hollow microneedles array with sonophoretic emitter has been used on pig skin to improve the
efficiency of drug delivery [173].
4. Discussion
MEMS and NEMS based microfluidic devices have many important characteristics that make them
attractive for biomedical applications. Microfluidic devices have the ability to control their physical
and chemical characteristics from a very small scale up to the nanometer range. These devices have
made it possible to meet critical medical needs such as nearly constant drug level at the site of action,
prevention of peak-valley fluctuations, site specific drug delivery, reduced side effects and increased
therapeutic effectiveness [4]. However, there are certain medical conditions for which constant drug
release pattern is not suitable. These conditions demand delayed release of drug. Such a release pattern
is known as pulsatile release. Recent research has shown that some diseases have a predictable cyclic
rhythm and the timing of drug release can significantly improve the outcome of a desired effect [219].
This condition requires release of drug as a pulse after a time delay. Some of the diseases where
pulsatile drug delivery devices are promising include duodenal ulcer, cardiovascular diseases, arthritis,
asthma, diabetes, neurological disorder, cancer, hypertension and hypercholesterolemia [4]. That is
why study of pulsatile flow is extremely important at small scales in microfluidic devices. Using
MEMS and NEMS technology, complex drug release patterns (such as simultaneous constant and
pulsatile release) can be achieved using integrated microfluidic systems. Microfluidic devices have
ability to control both release time and release rate. Micropumps and microneedles are essential
components for such biomedical systems. Micropumps are used for fluid transport and microneedles
provide interface between drug reservoir and patient body [4,13,6]. Material selection is a critical issue
Int. J. Mol. Sci. 2011, 12
in biomedical devices. Si has been widely used as material for such microfluidic devices, but
polymeric materials like PGA, PDMS, PMMA, PLLA, PLA, PC, etc. are replacing Si due to
biocompatibility, low cost, ease of fabrication and excellent structural properties. Various factors are
important during the selection of micropumps for particular biomedicine applications. Operating
voltage, pressure and flow rate of micropumps are critical issues to analyze the performance and
suitability of micropumps for certain medical applications. A schematic illustration of operating
voltages and flow rates of mechanical micropumps is shown in Figure 22.
Figure 22. Comparison of voltage versus flow rate for mechanical micropumps.
Piezoelectric and electromagnetic mechanical micropumps have been reported extensively for
microfluidic systems among the mechanical micropumps. The major limitation related to these types
of micropumps is very high operating voltage [224]. Electrostatic micropump is easy to fabricate on
integrated microfluidic systems but it also requires high operating voltage. The ICPF micropump has
an adequate flow rate at a relatively low operating voltage but with complex geometry. The schematic
illustration of operating voltages and flow rates of non-mechanical micropumps is shown in Figure 23.
Int. J. Mol. Sci. 2011, 12
Figure 23. Comparison of voltage versus flow rate for non-mechanical micropumps.
In non-mechanical micropumps, MHD micropump has gained more attention in recent years and
has been presented by many researchers for microfluidic systems. However, the electrochemical type
of micropump is more suitable for low voltage and high flow rate applications. Most micropumps and
microneedles have been reported in literature as an individual device for medical applications. Only a
few researchers have presented integrated devices [227]. Integration of micropumps and hollow
microneedles is a great challenge, but research on solid microneedles coated with nano-particles and
drugs has recently commenced in the biomedical field. However, hollow microneedles are more
attractive for fluid/drug transport. Microneedles can be integrated with micropumps or used as
stand-alone biomedical device. Various types of microneedles have been presented by different
researchers. In hollow microneedles, the side opened double lumen reservoir based microneedles are
more suitable for fluid transport. The pressure difference in the lumen regions is useful to avoid the
clogging effect. In solid microneedles, the sharper tip microneedles are more practical for drug
transport. Effectiveness of drug transport has also been presented in recent years by various researchers
using microneedles on mouse, pig, chicken and human. Various researchers have reported the structure
and fracture analyses of microneedles by applying force and pressure to predict the bending and failure
of microneedles. The schematic illustration of comparison of force and stress for microneedles has
been shown in Figure 24.
Int. J. Mol. Sci. 2011, 12
Figure 24. Comparison of force versus stress on microneedles.
5. Challenges and Future Aspects
In biomedical field, there are many challenges relating to the microfluidic devices (micropumps,
microneedles) such as design level issue, fabrication level issue, packaging level issues, use in
practical application, etc. At design and fabrication level, the most important issues and specifications
that must be fulfilled by the micropumps for particular applications are appropriate design for
maintaining a specific flow rate, control of back pressure, dosing accuracy, drugs resistive material
selection for fabrication, ease of fabrication, energy utilization, power supply at such small level,
bubble tolerance, durability and reliability. The most important issues relating to microneedles at
design and fabrication level are avoidance of clogging effect, suitable length, robustness, strength,
sharp tip to avoid pain, drugs resistance, less fabrication cost, reliability, biocompatibility, etc. Suitable
batch fabrication techniques need to be adopted to reduce cost of devices. Packaging of these devices
is very important consideration [229]. Packaging should be robust and strong enough to prevent
infection or damage of microfluidic devices. Simultaneously, the unintentional discharge of drug/fluid
during storage from the reservoir should be prevented. A protecting wrap may be possibly required to
secure such small size devices like micropumps and sharp tip microneedles. Mostly the micropumps
and microneedles reported in literature have been proposed as stand-alone devices. Integration of
micropump and microneedles is a great challenge that limits the use of these devices commercially for
biomedical applications. The final cost of these delivery devices should be affordable for the end
users/patients. The trend is now shifting towards the use of polymeric materials like PGA, PDMS,
PMMA, etc. for the fabrication of micropumps and microneeldes to overcome most of the above
described issues as these materials are cheap, biocompatible, exhibit excellent mechanical/chemical
Int. J. Mol. Sci. 2011, 12
properties, etc. [4,13,19,202]. Zosano Pharma [140] has developed the user friendly and simple TDD
patch system that can deliver vaccines, proteins, peptides and small molecules. 3 M has developed the
microneedles based transdermal system demonstrating good results on research level studies for
peptides, vaccines and protein [230]. Birchall et al. [231] conducted a survey to learn more about the
opinion of end users with regard to the convenience, efficacy and worth of microneedle technology.
Research on MEMS-based delivery devices shows that these devices are suitable for commercial
applications. However, the development of these devices is limited to research level due to some
factors such as investment, expertise for device development, marketing, awareness of public,
motivation, lack of collaboration between companies and research institutes, medical staff
training/recommendations, etc. Surveys, seminars, workshops, etc., need to be organized to promote
the benefits and convenience of using these delivery devices to end users. The availability of reliable
and manufacturable microfluidic devices will have a strong impact on the biomedicine field to meet
critical health care needs.
6. Conclusions
Fluid transport using microfluidic devices such as micropumps and microneedles is a relatively new
and attractive method that has many advantages. Microfluidic devices have received much more
attention in recent years due to their potential applications in the biomedicine field. Various types of
micropumps and microneedles structures using different materials like glass, silicon, metals, polymers,
etc., have been reported for biomedical applications, but Si has been mostly used as substrate material
for fabrication of microfluidic devices among other materials. Si is brittle and always some risk
involves for health care. Biocompatibility is very important for health and because of this reason the
trend is transferring towards polymeric materials. Most polymers, e.g., PGA, PDMS, PLA and PMMA,
are very suitable for biomedical devices due to their good biocompatibility, low cost, ease of
fabrication and excellent chemical and mechanical properties. Solid microneedles are easy to fabricate
and have more strength than hollow microneedles. However, the disadvantage of solid microneeldes is
the risk of fracture/breaking within the skin after being inserted. Drug particles can be coated in
restricted amounts. Hollow microneeldes are considered more suitable for TDD systems due to the
precise delivery of the desired amount of drug at a specific site with rapid action. ICPF and
electrostatic micropumps are considered suitable for drug/fluid delivery systems due to low operating
voltage and direct integration with electronic circuit respectively. The disadvantages of hollow
microneeldes and micropumps are complicated/costly fabrication, clogging effect, back pressure,
requirement of flow rate regulation, etc. Based on the presented literature review, the authors conclude
that MEMS based microfluidic devices for biomedical applications remain at the research level. Only a
few devices have been converted into commercial products due to some important issues like
complicated structure of microfluidic devices, difficulties in integration with other devices, investment,
expertise for device fabrication, marketing, public awareness, lack of collaboration between companies
and research institutes, medical staff training/recommendation and finally packaging. To present
microfluidic devices for practical applications in medical field, motivated researchers still need to
continue their work on the development of microfluidic devices.
Int. J. Mol. Sci. 2011, 12
References
Hsu, T. MEMS & Microsystem Design and Manufacturing, 1st ed.; McGraw-Hill: New York,
Hardt, S.; Schonfeild, F. Microfluidic Technologies for Maniaturized Analysis System, 1st ed.;
Springer: Berlin, Germany, 2010.
Ashraf, M.W.; Tayyaba, S.; Afzulpurkar, N.; Nisar, A. Fabrication and analysis of tapered tip
silicon microneedles for mems based drug delivery system. Sens. Transducer 2010, 122,
Ashraf, M.W.; Tayyaba, S.; Nisar, A.; Afzulpurkar, N.; Bodhale, D.W.; Lomas, T.; Poyai, A.;
Tuantranont, A. Design, fabrication and analysis of silicon hollow microneedles for transdermal
drug delivery system for Treatment of Hemodynamic Dysfunctions Cardiovasc. Eng. J. 2010, 3,
Prausnitz, M.R.; Langer, R. Transdermal drug delivery. Nat. Biotechnol. 2008, 26, 1261–1268.
Polla, D.L. BioMEMS Applications in Medicine. In Proceedings of International Symposium on
Micromechatronics and Human Science, Nagoya, Japan, 9–12 September 2001; pp. 13–15.
Shawgo, S.R.; Amy, C.; Grayson, R.; Yawen, L. Cima, J.M. BioMEMS for drug delivery. Curr.
Opin. Solid-State Mater. Sci. 2002, 6, 329–334.
Amy, C.; Grayson, R.; Shawgo, R.S.; Li, Y.; Cima, M.J. Electronic MEMS for triggered delivery.
Adv. Drug Deliv. Rev. 2004, 56, 173–184.
Staples, M.; Daniel, K.; Cima, M.J.; Langer, R. Application of micro and nano electromechanical
devices to drug delivery. Pharm. Res. 2006, 23, 847–863.
10. Laser, D.J.; Santiago, J.G. Areview of micropumps. J. Micromech. Microeng. 2004, 14, 35–64.
11. Woias, P. Micropumps-past, progress and future prospects. Sens. Actuators B 2005, 105, 28–38.
12. Tsai, N.C.; Sue, C.Y. Review of MEMS based drug delivery and dosing systems. Sens. Actuators A
2007, 134, 555–564.
13. Nisar, A.; Afzulpurkar, N.; Mahaisavariya, B.; Tuantranont, A. MEMS-based micropumps in
drug delivery and biomedical applications. Sens. Actuators B 2008, 130, 917–942.
14. Amirouche, F.; Zhou, Y.; Johnson. T. Current micropump technologies and their biomedical
applications. Microsyst. Technol. 2009, 15, 647–666.
15. Grayson, A.R.; Shawgo, R.S.; Johnson, A.M.; Flynn, N.T.; Yawen, L.; Cima, M.J.; Langer, R.
A BioMEMS review: MEMS technology for physiologically integrated devices. Proc. IEEE
2004, 92, 6–21.
16. Karman, S.; Ibrahim, F.; Soin, N. A review of MEMS drug delivery in medical application,
Biomed 06. Proc. IFMBE 2007, 15, 312–315.
17. Bao-jian, X.; Qing-hui, J.; Jian-long, Z. An review of MEMS-Based microneedles technology
developments. Micronanoelectron. Technol. 2005, 4, doi:1671-4776.0.2005-04-002.
18. Khanna, P.; Storm, J.A.; Malone, J.I.; Bhansali, S. Microneedle-Based Automated Therapy for
Diabetes Mellitus. J. Diabetes Sci. Technol. 2008, 2, 1122–1129.
19. Sachdeva, V.; Banga, A.K. Microneedles and their applications. Recent Pat. Drug Deliv. Formul.
2011, 5, 95–132.
Int. J. Mol. Sci. 2011, 12
20. Stemme, E.; Stemme, G. A valveless diffuser/nozzle based fluid pump. Sens. Actuators A 1993,
39, 159–167.
21. White, F.M. Fluid Mechanics, International Student Edition; McGraw Hill Inc.: Columbus, OH,
USA, 1979; pp. 161–162.
22. Van Lintel, H.T.G.; Van de Pol, F.C.M.; Bouwstra, S. A piezoelectric micropump based on
micromachining of silicon. Sens. Actuators A 1988, 15, 153–168.
23. Esashi, M.; Shoji, S.; Nakano, A. Normally close microvalve and micropump fabricated on a
silicon wafer. In Proceedings of IEEE Micro Electro Mechanical Systems, An Investigation of
Micro Structures, Sensors, Actuators Machines and Robots, Salt Lake City, UT, USA, 20–22
February 1989; pp. 29–34.
24. Olsson, A.; Stemme, G.; Stemme, E. A valve-less planar fluid pump with two pump chambers,
Sens. Actuators A 1995, 47, 549–556.
25. Koch, M.; Harris, N.; Evans, A.G.R.; White, N.M.; Brunnschweiler, A. A novel micromachined
pump based on thick film piezoelectric actuation. Sens. Actuators A 1998, 70, 98–103.
26. Schabmueller, C.G.J.; Koch, M.; Mokhtari, M.E.; Evans, A.G.R.; Brunnschweiler, A.; Sehr, H.
Self-aligning gas/liquid micropump. J. Micromech. Microeng. 2002, 12, 420–424.
27. Feng, G.H.; Kim, E.S. Piezoelectrically actuated dome-shaped diaphragm micropump.
J. Microelectromech. Syst. 2005, 14, 92–199.
28. Geipel, A.; Doll, A.; Goldschmidtboing, F.; Jantscheff, P.; Esser, N.; Massing, U.; Woias, P.
Pressure independent micropump with piezoelectric valves for low flow drug delivery systems.
In Proceedings of 19th IEEE International Conference
Istanbul, Turkey, 22–26 January 2006; pp. 786–789.
29. Trenkle, F.; Haeberle, S.; Zengerle, R. Normally-closed peristaltic micropump with re-usable
actuator and disposable fluidic chip. Procedia Chem. 2009, 1, 1515–1518.
30. Johari, J.; Yunas, J.; Yeopmajlis, B. Piezoeletric Micropump for Drug Delivery System
Fabricated Using Two Optical Masks. Adv. Mat. Res. 2009, 74, 279–282.
31. Wang, C.; Leu, T.; Sun, J. Asymmetrical flow effect applied to pumping performance of simple
duct channel. Sens. Actuators A 2009, 155, 203–209.
32. Ali, M. Y.; Kuang, C.; Khan, J.; Wang, G. A dynamic piezoelectric micropumping phenomenon.
Microfluid. Nanofluid. 2010, 9, 385–396.
33. Liu, G.; Shen, C.; Yang, Z.; Cai, X.; Zhang, H. A disposable piezoelectric micropump with high
performance for closed-loop insulin therapy system. Sens. Actuators A 2010, 163, 291–296.
34. Judy, J.W.; Tamagawa, T.; Polla, D.L. Surface-machined Micromechanical Membrane Pump. In
Proceedings of the IEEE Micro Electro Mechanical systems, Nara, Japan, 30 January–2 February
1991; pp. 182–186.
35. Zengerle, R.; Richter, A.; Sandmaier, H. A Micro Membrane Pump with Electrostatic Actuation.
In Proceedings of Micro Electro Mechanical Systems 1992, An Investigation of Micro Structures,
Sensors, Actuators, Machines and Robot. IEEE, Travemunde, Germany, 4–7 February 1992;
36. Cabuz, C.; Herb, W.R.; Cabuz, E.I.; Lu, S.T. The dual diaphragm pump. In Proceedings of the
14th IEEE International Conference on Micro Electro Mechanical Systems, Interlaken,
Switzerland, 21–25 January 2001; pp. 519–522.
Int. J. Mol. Sci. 2011, 12
37. Machauf, A.; Nemirovsky, Y.; Dinnar, U. A membrane micropump electrostatically actuated
across the working fluid. J. Micromech. Microeng. 2005, 15, 2309–2316.
38. Astle, A.A.; Kim, H.S.; Bernal, L.P.; Najafi, K.; Washabaugh, P.D. Theoretical and experimental
performance of a high frequency gas micropump. Sens. Actuators A 2006, 134, 245–256.
39. Lee, S.; Yee, S.Y.; Besharatian, A.; Kim, H.; Bernal, L.P.; Najafi, K. Adaptive gas pumping by
colntrolled timing of active microvalves in peristaltic micropumps. In Proceedings of
Transducers, Denver, CO, USA, 21–25 June 2009.
40. Liu, W. Research on Electrostatic Micropump Pull-in Phenomena Based On Reduced Order
Model. In Proceedings of International Conference on Intelligent Computation Technology and
Automation, Changsha, China, 11–12 May 2010.
41. L.; R.; Zhou, Z.; Ren, J. Modeling of a Micropump Membrane with Electrostatic
Actuator. In Proceedings of 2nd International Conference on Advanced Computer Control
(ICACC), Shenyang, China, 27–29 March 2010.
42. Van de Pol, F.C.M.; van Lintel, H.T.G.; Elwenspoek, M.; Fluitman, J.H.J. A thermopneumatic
micropump based on microengineering techniques. Sens. Actuators A 1990, 21, 198–202.
43. Jeong, O.C.; Yang, S.S. Fabrication and test of a thermopneumatic micropump with a corrugated
p diaphragm, Sens. Actuators 2000, 83, 249–255.
44. Cooney, C.G.; Towe, B.C. A thermopneumatic dispensing micropump. Sens. Actuators A 2004,
116, 519–524.
45. Kim, J.H.; Na, K.H.; Kang, C.J.; Kim, Y.S. A disposable thermopneumatic actuated micropump
stacked with PDMS layers and ITO coated glass, Sens. Actuators A 2005, 120, 365–369.
46. Jeong, O.C.; Konishi, S. Fabrication of a peristaltic micro pump with novel cascaded actuators.
J. Micromech. Microeng. 2008, 18, 025022.
47. Chia, B.T.; Liao, H.; Yang, Y. A novel thermo-pneumatic peristaltic micropump with low
temperature elevation on working fluid. Sens. Actuators A 201086–93.
48. Tan, H.Y.; Loke, W.K.; Nguyen, N. A reliable method for bonding polydimethylsiloxane (PDMS)
to polymethylmethacrylate (PMMA) and its application in micropumps. Sens. Actuators B 2010,
151, 133–139.
49. Zheng, W.; Ahn, C.H. A Bi-directional Magnetic Micropump on a Silicon Wafer. In Proceedings
of Technical Digest Solid-State Sensor and Actuator Workshop 1996, Hilton Head Island, SC,
USA, 3–6 June 1996.
50. Bohm, S.; Olthuis, W.; Bergveld, P. A plastic micropump constructed with conventional
techniques and materials. Sens. Actuators A 1999, 77, 223–228.
51. Gong, Q.; Zhou, Z.; Yang, Y.; Wang, X. Design, optimization and simulation on
microelectromagnetic pump. Sens. Actuators A 2000, 83, 200–207.
52. Yamahata, C.; Lotto, C.; Al Assaf, E.; Gijs, M.A.M. A PMMA valveless micropump using
electromagnetic actuation. Microfluid. Nanofluid. 2005, 1, 197–207.
53. Su, Y.; Chen, W.; Cui, F.; Zhang, W. Analysis and fabrication process of an electromagnetically
actuated valveless micropump with two parallel flexible diaphragms. Proc. IMechE 2005, 219,
54. Balaji, G.; Singh, S.; Ananthasuresh, G.K. Electro-magnetically Actuated Minute Polymer Pump
Fabricated using Packaging Technology. J. Phys. 2006, 34, 258–263.
Int. J. Mol. Sci. 2011, 12
55. Yu-feng, S.U.; Wen-yuan, C.; Feng, C.; Wei-ping, Z. Design and fabrication process of
electromagnetically actuated valveless micropump with two parallel flexible diaphragms.
J. Shanghai Univ. 2007, 11, 79–83.
56. Shen, M.; Yamahata, C.; Gijs, M.A.M. Miniaturized PMMA ball-valve micropump with
cylindrical electromagnetic actuator. Microelectr. Eng. 2008, 85, 1104–1107.
57. Al-Halhouli, A.T.; Kilani, M.I.; Büttgenbacha, S. Development of a novel electromagnetic pump
for biomedical applications. Sens. Actuators A 2010, 162, 172–176.
58. Zhan, C.; Lo, T.; Liu, L.P. A silicon membrane micropump with integrated bimetallic actuator.
Chin. J. Electron. 1996, 5, 29–35.
59. Zou, J.X.; Ye, Y.Z.; Zhou, Y.; Yang, Y. A Novel Thermally Actuated Silicon Micropump.
In Proceedings of International Symposium on Micromechatronics and Human Science, Nagoya,
Japan, 5–8 October 1997.
60. Pang, J.; Zou, Q.; Tan, Z.; Qian, X.; Liu, L.; Li, Z. The Study of Single Chip Integrated
Microfluidic System. In Proceedings of 5th International Conference on Solid State and
Integrated Circuit Technology, Beijing, China, 21–23 October 1998; pp. 895–898.
61. Yang, Y.; Zhaoying, Z.; Xiongying, Y.; Xiaoning, J. Bimetallic Thermally Actuated Micropump.
In Proceedings of International Mechnical Engineering Congross and Exposition (ASME 1996),
Atlanta, GA, USA, 17–22 November 1996.
62. Guo, S.; Nakamura, T.; Fukuda, T.; Oguro, K. Design and Experiments of Micropump Using
ICPF Actuator. In Proceedings of 7th International IEEE Symposium on Micro Machine and
Human Science, Nagoya, Japan, 2–4 October 1996; pp. 235–240.
63. Tadokoro, S.; Yamagami, S.; Ozawa, M. Soft Micromanipulation Device with Multiple Degrees
of Freedom Consisting of High Polymer Gel Actuators. In Proceedings of IEEE International
Conference on Microelectromechanical Systems, Orlando, FL, USA, 17–21 January 1999;
64. Guo, S.; Asaka, K. Polymer Based New Type of Micropump for Biomedical Applications.
In Proceedings of IEEE Conference on Robotics & Automation, Taipei, Taiwan, 14–19
September 2003; pp. 1830–1835.
65. Nguyen, T.T.; Goo, N.S.; Nguyen, V.K.; Yoo, Y.; Park, S. Design, fabrication, and experimental
characterization of a flap valve IPMC micropump with a flexibly supported diaphragm. Sens.
Actuators A 2008, 141, 640–648.
66. Chen, Z.; Kwon, K.; Tan, X. Integrated IMPC/PVDF sensory actuator and its validation in
feedback control. Sens. Actuators A 2008, 144, 231–241.
67. Fang, T.; Tan, X. A novel diaphragm micropump actuated by conjugated polymer petals:
Fabrication, modeling, and experimental results. Sens. Actuators A 2010, 158, 121–131.
68. Sim, W.Y.; Yoon, H.J.; Jeong, O.C.; Yang, S.S. A phase change type of micropump with
aluminum flap valves. J. Micromech. Microeng. 2003, 13, 286–294.
69. Boden, R.; Lehto, M.; Simu, U.; Thornell, G.; Hjort, K.; Schweitz, J.A. A Polymeric Paraffin
Micropump with Active Valves for High Pressure Microfluidics. In Proceedings of 13th
International Conference on Solid State Sensors, Actuators and Microsystems, Seoul, Korea, 5–9
June 2005; pp. 201–204.
Int. J. Mol. Sci. 2011, 12
70. Sim, W.Y.; Lee, S.W.; Yang, S.S. The Fabrication and Test of a Phase-change Type Micropump,
2008. Available online: http://click.ndsl.kr/servlet/LinkingFullTextView?service_code=04&dbt
=JAKO&cn=JAKO200011919587765 (accessed on 20 February 2011).
71. Benard, W.L.; Kahn, H.; Heuer, A.H.; Huff, M.A. Thin-film shapememory alloy actuated
micropumps. J. Microelectromech. Syst. 1998, 7, 245–251.
72. Makino, E.; Mitsuya, T.; Shibata, T. Fabrication of TiNi shape memory micropump. Sens.
Actuators A 2001, 88, 256–262.
73. Xu, D.; Wang, L.; Ding, G.; Zhou, Y.; Yu, A.; Cai, B. Characteristics and fabrication of NiTi/Si
diaphragm micropump. Sens. Actuators A 2001, 93, 87–92.
74. Shuxiang, G.; Fukuda, T. SMA Actuator Based Novel Type of Micropump for Biomedical
Application. In Proceedings of IEEE International Conference
Takamatsu, Japan, 26 April–1 May 2004; Volume 2, pp. 1616–1621.
75. Zhang, H.J.; Qiu, C.J. A TiNiCu thin film micropump made by magnetron Co-sputtered method.
Mat. Trans. 2006, 47, 532–535.
76. Setiawan, M.A. The performance evaluation of SMA Spring as actuator for gripping
manipulation. J. Teknik Elektro 2007, 7, 110–120.
77. Zhu, M.; Kirby, P.; Wacklerle, M.; Herz, M.; Richter, M. Optimization design of multi-material
micropump using finite element method. Sens. Actuators A 2009, 149, 130–135.
78. Kang, J.; Auner, G.W. Simulation and verification of a piezoelectrically actuated diaphragm for
check valve micropump design. Sens. Actuators A 2011, in press.
79. Shen, M.; Dovat, L.; Gijs, M.A.M. Magnetic active-valve micropump actuated by a rotating
magnetic assembly. Sens. Actuators B 2009, 154, 52–58.
80. Teymoori, M.M.; Abbaspour-Sani, E. Design and simulation of a novel electrostatic peristaltic
micromachined pump for drug delivery applications. Sens. Actuators A 2005, 117, 222–229.
81. Zeng, S.; Chen, C.H.; Mikkelsen, J.C.; Santiago, J.G. Fabrication and characterization of
electroosmotic micropumps. Sens. Actuators B 2001, 79, 107–114.
82. Takemori, Y.; Horiike, S.; Nishimoto, T.; Nakanishi, H.; Yoshida, T. High Pressure Electroosmotic
Pump Packed with Uniform Silica Nanosphers. In Proceedings of 13th International Conference
on Solid State Sensors, Actuators and Microsystems, Seoul, Korea, 5–9 June 2005.
83. Hu, J.S.; Chao, C.Y.H. Numerical study of electroosmotic (EO) flow in microfabricated EO
pump with overlapped electrical double layer (EDL). Int. J. Refrig. 2007, 30, 290–298.
84. Good, B.T.; Bowman, C.N.; Davis, R.T. A water-activated pump for portable microfluidic
applications. J. Colloid Interface Sci. 2007, 305, 239–249.
85. Ryu, W.H.; Huang, Z.; Prinz, F.B. Goodman, S.B. Fasching, R. Biodegradable microosmotic
pump for long term and controlled release of basic fibroblast growth factor. J. Control. Release
2007, 124, 98–105.
86. Yairi, M.; Richter, C. Massively parallel microfluidic pump. Sens. Actuators A 2007, 137, 350–356.
87. Borowsky, J.; Lu, Q.; Collins, G.E. High pressure electroosmotic pump based on a packed bed
planar microchip. Sens. Actuators B 2008, 131, 333–339.
88. Wang, X.; Wang, S.; Gendhar, B.; Cheng, C.; Byun, C.K.; Li, G.; Zhao, M.; Liu, S. Electroosmotic
pumps for microflow analysis. Trends Anal. Chem. 2009, 28, 64–74.
Int. J. Mol. Sci. 2011, 12
89. Yun, K.S.; Cho, I.J.; Bu, J.U.; Kim, C.J.; Yoon, E. A surface tension driven micropump for low
voltage and low power operations. J. MEMS 2002, 11, 454-461.
90. Hoshino, K.; Triteyaprasert, S.; Matsumoto, K.; Shimoyama, I. Electrowetting-based pico-liter
liquid actuation in a glass-tube microinjector. Sens. Actuators A 2004, 114, 473–477.
91. Colgate, E.D.; Matsumoto, H. An investigation of electrowetting based microactuation. J. Vac.
Sci. Technol. A 1990, 4, 3625–3633.
92. Chang, J.; Choi, D.Y.; Han, S.; Pak, J.J. Driving characteristics of the electrowetting-on-dielectric
device using atomic-layer-deposited aluminum oxide as the dielectric. Microfluid. Nanofluid.
2010, 8, 269–273.
93. Suzuki, H.; Yoneyama, R. Areversible electrochemical nanosyringe pump and some
considerations to realize low power consumption. Sens. Actuators B 2002, 86, 242–250.
94. Suzuki, H.; Yoneyama, R. Integrated microfluidic system with electrochemically actuated
on-chip pumps and valves. Sens. Actuators B 2003, 96, 38–45.
95. Yoshimi, Y.; Shinoda, K.; Mishima, M.; Nakao, K.; Munekane, K. Development of an artificial
synapse using an electrochemical micropump. J. Artif. Organs 2004, 7, 210–215.
96. Kim, J.H.; Laua, K.T.; Shepherd, R.; Wu, Y.; Wallace, G.; Diamond, D. Performance
characteristics of a polypyrrole modified polydimethylsiloxane (PDMS) membrane based
microfluidic pump. Sens. Actuators A 2008, 148, 239–244.
97. Effenhauser, C.S.; Harttig, H.; Kramer, P. An evaporation-based disposable micropump concept
for continuous monitoring applications. Biomed. Microdev. 2002, 4, 27–33.
98. Namasivayam, V.; Larson, R.G.; Burke, D.T.; Burns, M.A. Transpirationbased micropump for
delivering continuous ultra low flow rates. J. Micromech. Microeng. 2003, 13, 261–271.
99. Guan, Y.; Xua, Z.; Dai, J.; Fang, Z. The use of a micropump based on capillary and evaporation
effects in a microfluidic flow injection chemiluminescence system. Talanta 2006, 68, 1384–1389.
100. Heuck, F.; Hug, T.; Akiyama, T.; Frederix, P.L.T.M.; Engel, A.; Meister, A.; Heinzelmann, H.;
de Rooij, N.F.; Staufer, U. Evaporation based micro pump integrated into a scanning force
microscope probe. Microelectr. Eng. 2008, 85, 1302–1305.
101. Tsai, J.H.; Lin, L.; A Thermal Bubble Actuated Micro Nozzle-diffuser Pump. In Proceedings of
14th IEEE International Conference Microelectromechanical Systems, Interlaken, Switzerland,
21–25 January 2001; pp. 409–412.
102. Tsai, J.H.; Lin, L. Active microfluidic mixture and gas bubble filter driven by thermal bubble
micropump. Sens. Actuators A 2002, 97-98, 665–671.
103. Lew, K.S.F.; Klaseboer, E.; Khoo, B.C. A collapsing bubble-induced micropump: An
experimental study. Sens. Actuators A 2007, 133, 161–172.
104. Jung, J.Y.; Kwak, H.Y. Fabrication and testing of bubble powered micropumps using embedded
microheater. Microfluid. Nanofluid. 2007, 3, 161–169.
105. Cheng, C.M.; Liu, C.H. An Electrolysis-Bubble-Actuated micropump based on the roughness
gradient design of hydrophobic surface. J. Microelectromech. Syst. 2007, 16, 1095–1105.
106. Chan, S.C.; Chen, C.R.; Liu, C.H. A bubble-activated micropump with high-frequency flow
reversal. Sens. Actuators A 2010, 163, 501–509.
107. Jang, J.; Lee, S.S. Theoretical and experimental study of MHD (magnetohydrodynamic)
micropump. Sens. Actuators A 2000, 80, 84–89.
Int. J. Mol. Sci. 2011, 12
108. Zhong, J.; Yi, M.; Bau, H.H. Magneto Hydrodynamic (MHD) pump fabricated with ceramic
tapes. Sens. Actuators A 2002, 96, 59–66.
109. Eijkel, J.C.T.; Dalton, C.; Hayden, C.J.; Burt, J.P.H.; Manz, A. A circular ac magnetohydrodynamic
micropump for chromatographic applications. Sens. Actuators B 2003, 92, 215–221.
110. Patel, V.; Kassegne, S.K. Electroosmosis and thermal effects in Magnetohydrodynamic (MHD)
micropumps using 3D MHD equations. Sens. Actuators B 2007, 122, 42–52.
111. Duwairi, H.M.; Abdullah, M. Numerical Computation of Fluid Flow in a Magnetohydrodynamic
Micropump. Turkish J. Eng. Env. Sci. 2008, 32, 1–5.
112. Kang, H.; Choi, B. Development of the MHD micropump with mixing function. Sens. Actuators A
2010, 165, 439–445.
113. Moroney, R.M.; White, R.M.; Howe, R.T. Microtransport induced by ultrasonic lamb waves.
Appl. Phys. Lett. 1991, 59, 774–776.
114. Nguyen, N.T.; White, R.M. Design and optimization of an ultrasonic flexural plate wave
micropump using numerical simulation. Sens. Actuators A 1999, 77, 229–236.
115. Nguyen, N.T.; Meng, A.H.; Black, J.; White, R.M. Integrated flow sensor for insitu measurement
and control of acoustic streaming in flexural plate wave micropumps. Sens. Actuators A 2000,
79, 115–121.
116. Meng, A.H.; Nguyen, N.T.; White, R.M. Focused flow micropump using ultrasonic flexural plate
waves. Biomed. Microdevices 2000, 2–3, 169–174.
117. D.R. Microfluidic circulatory flows induced by
resonant vibration of diaphragms.–148.
118. Singh, R.; Bhethanabotla, V.R. Enhancement in Ultrasonic Micro-Transport Using Focused
Inter-Digital Transducers in a Surface Acoustic Wave Device: Fluid-Structure Interaction Study.
In Proceedings of IEEE Sensors, Christchurch, New Zealand, 25–28 October 2009.
119. Richter, A.; Sandmaier, H. An Electrohydrodynamic Micropump. In Proceedings of IEEE
Microelectromechanical Systems, Napa Valley, CA, USA, 11–14 February 1990; pp. 99–104.
120. Fuhr, G.; Hagedorn, R.; Muller, T.; Benecke, W.; Wagner, B. Pumping of Water Solutions in
Microfabricated Electrohydrodynamic Systems. In Proceedings of IEEE
MEMS, Travemunde, Germany, 4–7 February 1992; pp. 25–30.
121. Darabi, J.; Ohadi, M. M.; DeVoe, D. An Electrohydrodynamic polarization micropump for
electronic cooling. J. Microelectromech. Syst. 2001, 10, 98–106.
122. Darabi, J.; Rada, M.; Ohadi, M.; Lawler, J. Design, fabrication and testing of an
electrohydrodynamic ion drag micropump. J. Microelectromech. Syst. 2002, 11, 684–690.
123. Yang, L.J.; Wang, J.M.; Huang, Y.L. The micro ion drag pump using indium-tin-oxide (ITO)
electrodes to resist aging. Sens. Actuators A 2004, 111, 118–122.
124. Lin, C.W.; Jang, J.Y. 3D numerical micro-cooling analysis for an electrohydrodynamic
micro-pump. Sens. Actuators A 2005, 122, 167–176.
125. Darabi, J.; Rhodes, C. CFD modeling of an ion-drag micropump. Sens. Actuators A 2006, 127,
126. Singhal, V.; Garimella, S.V. Induction electrohydrodynamics micropump for high heat flux
cooling. Sens. Actuators A 2007, 134, 650–659.
Int. J. Mol. Sci. 2011, 12
127. Wakui, D.; Imai, N.; Nagaura, Y.; Sato, H.; Sekiguchi, T.; Konishi, S.; Shoji, S.; Homma, T.;
EHD Micropump using Pyrolyzed Polymer 3-D Carbon Mesh Electrodes. In Proceedings of
22nd IEEE International Conference on
128. Litster, S.; Suss, M.E. Santiago, J.G. A two-liquid electroosmotic pump using low applied
voltage and power. Sens. Actuators A 2010, 163, 311–314.
129. Xu, Z.; Yang, C.; Liua, C.; Zhou, Z.; Fang, J.; Wang, J.; An osmotic micro-pump integrated on a
microfluidic chip for perfusion cell culture. Talanta 2010, 80, 1088–1093.
130. Lim, S.; Choi, B. A study on the MHD (magnetohydrodynamic) micropump with side-walled
electrodes. J. Mech. Sci. Technol. 2009, 23, 739–749.
131. Luginbuhl, P. Microfabricated lamb wave device based on PZT sol-gel thin film for mechanical
transport of solid particles and liquids. J. Microelectromech. Syst. 1997, 6, 337–345.
132. Campbell, P.K.; Jones, K.E.; Huber, R.J.; Horch, K.W.; Normann, R.A. A silicon-based three
dimensional neural interface: Manufacturing processes for an intracortical electrode array. IEEE
Trans. Biomed. Eng. 1991, 38, 758–768.
133. Bodhale, D.W.; Nisar, A.; Afzulpurkar, N. Structural and microfluidic analysis of hollow side-open
polymeric microneedles for transdermal drug delivery applications. Microfluid. Nanofluid. 2010,
8, 373–392.
134. Najafi, K.; Wise, K. An implantable multielectrode array with on-chip signal processing. IEEE J.
Solid-State Circ 1986, 21, 1035–1044.
135. Chen, J.; Wise, K.; Hetke, J.; Bledsoe, S. A multichannel neural probe for selective chemical
delivery at the cellular level. IEEE Trans. Biomed. Eng. 1997, 44,760–769.
136. Bai, Q.; Wise, K.; Anderson, D. A high-yield microassembly structure for three dimensional
microelectrode arrays. IEEE Trans. Biomed. Eng. 2000, 47, 281–289.
137. The World Book Encyclopedia. Hypodermic injection. The world book encyclopedia. World
Book Chic 1999, 9, 480–481.
138. McAllister, D.V.; Allen, M.G.; Prausnitz. M.R. Microfabricated microneedles for gene and drug
delivery. Annu. Rev. Biomed. Eng. 2000, 2, 289–313.
139. Lin, L.; Pisano, A. Silicon processed microneedles. J. Microelectromech. Syst. 1999, 8, 78–84.
140. Zosano Pharma. Available online(accessed on 27 April 2011).
141. Ameri, M.; Daddona, P.; Maa, Y. Demonstrated solid-state stability of parathyroid hormone
PTH(1-34) coated on a novel transdermal microprojection delivery system. Pharm. Res. 2009,
26, 2454–2463.
142. Ameri, M.; Daddona, P.; Maa, Y. Parathyroid hormone PTH(1–34) formulation that enables
uniform coating on a novel transdermal microprojection delivery system. Pharm. Res. 2009, 27,
143. Dizon, R.; Han, H.; Russell, A.; Reed, M. An ion milling pattern transfer technique for
fabrication of three-dimensional micromechanical structures. J. Microelectromech. Syst. 1993, 2,
144. Henry, S.; McAllister, D.; Allen, M.; Prausnitz, M. Microfabricated microneedles: A novel
approach to transdermal drug delivery. J. Pharm. Sci. 1998, 87, 922–925.
Int. J. Mol. Sci. 2011, 12
145. Chun, K.; Hashiguchi, G.; Toshioyoshi, H.; Pioufle, B.; Ishikawa, J.; Murakami, Y.; Tamiya, E.;
Kikuchi, Y.; Fujita, H. DNA injection into cell conglomerates by micromachined hollow
microcapillary arrays. In Proceedings of Digital Transducers, 10th International Conference
Solid-State Sensors and Actuators, Sendai, Japan, 7–10 June 1999; pp. 41–47.
146. Oka, K.; Aoyagi, S.; Arai, Y.; Isono, Y.; Hashiguchi, G.; Fujita, H. Fabrication of a microneedle
for a trace blood test. Sens. Actuators A 2002, 97-98, 478–485.
147. Brazzle, J.; Papautsky, I.; Frazier, B. Hollow metallic micromachined needle arrays. J. Biomed.
Devices 2000, 2, 197–205.
148. Chandrasekaran, S.; Brazzle, J.D.; Frazier, A.B. Surface micromachined metallic microneedles.
J. Microelectromech. Syst. 2003, 12, 289–295.
149. Chandrasekaran, S.; Frazier, A.B. Characterization of surface micromachined metallic
microneedles. J. Microelectromech. Syst. 2003, 12, 281–288.
150. Stoeber, B.; Liepmann, D. Design, Fabrication and Testing of a MEMS Syringe. In Proceedings
of Solid-State Sensors, Actuator and Microsystems Workshop, Hilton Head Island, SC, USA, 2–6
June 2002; pp. 77–80.
151. Hashmi, S.; Ling, P.; Hashmi, G.; Reed, M.; Gaugler, R.; Trimmer, W. Genetic transformation of
nematodes using arrays of micromechanical piercing structures. Biotechniques 1995, 19, 766–770.
152. Narayan, J.R.; Doraiswamy, A.; Chrisey, D.B.; Chichkov, B.N. Medical prototyping using two
photon polymerization. Materialstoday 2010, 12, 43–48.
153. Wu, Y.; Microneedle-based drug delivery: Studies on
delivery parameters and biocompatibility. Biomed. Microdevices 2008, 10, 601–610.
154. Coulman, S.A.; C.; A.; P.; C.;
J.C. Microneedle mediated delivery of nanoparticles into human skin. Int. J. Pharm.
2009, 366, 190–200.
155. Haq, M.I.; E.; D.N.; M.; C.; A.;
J.C. Clinical administration of microneedles: skin puncture, pain and sensation. Biomed.
Microdevices 2009, 11, 35–47.
156. Oh, J.H.; ; ;
Influence of the delivery systems using a microneedle array
on the permeation of a hydrophilic molecule, calcein, Eur. J. Pharm. Biopharm. 2008, 69,
157. Stoeber, B.; Liepmann, D. Fluid Injection Through Out-of-plane Microneedles. In Proceedings
of 1st Annual International Conference on Microtechnologies in Medicine and Biology,
Berkeley, CA, USA, 12–14 October 2000.
158. Griss, P.; Side-opened out-of-plane microneedles for microfluidics transdermal
liquid transfer. J Microelectromech. Syst. 2003, 12, 296–301.
In-plane single-crystal-silicon microneedles for minimally invasive microfluidic systems. Sens.
Actuators A 2004, 114, 276–284.
160. Wilke, N.; Hibert, C.; O'Brien, J.; Morrissey, A. Silicon microneedle electrode array with
temperature monitoring for electroporation. Sens. Actuators A 2005, 123–124, 319–325.
Int. J. Mol. Sci. 2011, 12
161. Choi, J.WS.H. Insertion Force Estimation of
Various Microneedle Array-type Structures Fabricated by a Microstereolithography Apparatus.
In Proceedings of International Joint Conference SICE-ICASE, Busan, Korea, 18–21 October
2006; pp. 3678–3681.
162. Shibata, T.;
Fabrication and mechanical characterization of microneedle array for cell surgery. In
Proceedings of
Lyon, France, 10–14 June 2007; pp. 719–722.
163. Runyam, W.R.; Bean, K.E. Semiconductor Integrated Circuit Processing Technology; Addison-
Wisley: New York, NY, USA, 1990.
164. Ambrose, C.G.; Clanto, T.O. Bioabsorbable implants, review of clinical experience in orthopedic
surgery. Ann. Biomed. Eng. 2004, 32, 171–177.
165. Park, J.H.; Allen, M.G.; Prausnitz, M.R. Biodegradable polymer microneedles: fabrication,
mechanics and transdermal drug delivery. J. Control. Release 2005, 104, 51–66.
166. Aoyagi, M. Biodegradable polymer needle with various tip angles and
consideration on insertion mechanism of mosquito's proboscis. Sens. Actuators A 2008, 143,
20–28.
167. Jiang, Intrascleral drug delivery to the eye
using hollow microneedles. Pharm. Res. 2009, 26, 395–403.
168. Parker, E.R.; Rao, M.P.; Turner, K.L.; Meinhart, C.D.; MacDonald, N.C.; Bulk micromachined
Titanium microneedles. J. Microelectromech. Syst. 2007, 16, 2, 289–295.
169. Yoshida, K.; I.; M.; M. Implantation mechanics of tungsten
microneedles into peripheral nerve truks. Med. Bio. Eng. Comput. 2007, 45, 413–420.
170. Ashraf, M.W.; Tayyaba, S.; Afzulpurkar, N.; Nisar, A. Punyasai, C.; Saejok, K.; Supadech, J.;
Atthi, N.; Hruanun, C.; Poyai, A. Optimization of Fabrication Process for MEMS based
Microneedles Using ICP Etching Technology. In Proceedings of 6th international Conference on
MEMS, Nano and Smart System (ICMENS), Changsha, China, 14–15 December 2010;
171. Tsuchiya, K.; Development of Blood Extraction
System for Health Monitoring System. Biomed. Devices 2005, 7, 347–353.
172. Ding, Z.; Verbaan, F.J.; Bivas-Benita, M.;D.G.;
G.; J.A. Microneedles arrays for the transcutaneous immunization of
diphtheria and influenza in BALB/c mice. J. Control. Release 2009, 136, 71–78.
173. Chen, B.; Wei, J.; Iliescu, C. Sonophoretic enhanced microneedles array (SEMA)-improving the
efficiency of transdermal drug delivery. Sens. Actuators B 2010, 145, 54–60.
174. Zhang, W.; Gao, J.; Zhu, Q.; Zhang, M.; Ding, X.; Wang, X.; Hou, X.; Fan, W.; Ding, B.;
Wu, X.; Wang, X.; Gao, S. Penetration and distribution of PLGA nanoparticles in the human skin
treated with microneedles. Int. J. Pharm. 2010, 402, 205–212.
175. Zhang, P.; Dalton, C.; Jullien, G.A. Design and fabrication of MEMS based microneedle arrays
for medical applications. Microsyst. Technol. 2009, 15, 1073–1082.
176. Yu, L.M.; Tay, F.E.H.; Guo, D.G.; Xu, L.; Yap, K.L. A microfabricated electrode with Hollow
microneedles for ECG measurement. Sens. Actuators A 2009, 151, 17–22.
Int. J. Mol. Sci. 2011, 12
177. Chen, B.; Wei, J.; Tay, F.E.H. Silicon microneedle array with biodegradable tip for transdermal
drug delivery. Microsyst. Technol. 2008, 14, 1015–1019.
178. Roxhed, N.; Griss, P.; Stemme, G. Membrane-seald hollow microneedles and related
administration schemes for transdermal drug delivery. Biomed. Microdevices 2008, 10, 271–279.
179. Bhandari, R.; Negi, S.; Solzbacher, F. A novel mask-less method of fabricating high aspect ratio
microneedles for blood sampling. In Proceedings of IEEE 58th Electronic Components and
Technology Conference, Lake Buena Vista, FL, USA, 27–30 May 2008; pp. 1306–1309.
180. Donnelly, R.F.; Morrow, D.I.J.; McCarron, P.A.; Woolfson, A.D.; Morrissey, A.; Juzenas, P.;
Juzeniene, A.; Lani, V.; McCarthy, H.O.; Moan, J. Microneedle-mediated Intradermal delivery
of 5-aminolevulinic acid: Potential for enhanced topical photodynamic therapy. J. Control.
Release 2008, 129, 154–162.
181. Lee, J.W.; Park, J.H.; Prausnitz, M.R. Dissolving microneedles for transdermal drug delivery.
Biomaterials 2008, 29, 2113–2124.
182. Park, J.H.; Choi, S.; Seo, S.; Choy, Y.B.; Prausnitz, M.R. A microneedle roller for transdermal
drug delivery. Eur. J. Pharm. Biopharm. 2010, 76, 282–289.
183. Gomaa, Y.A.; Morrow, D.I.J.; Garland, M.J.; Donnelly, R.F.; El-Khordagui, L.K. Effects of
microneedle length, densty, insertion time and multiple applications on human skin barrier
function: Assessments by transdermal water loss. Toxicol. in Vitro 2010, 24, 1971–1978.
184. Donnelly, R.F.; Morrow, D.I.J.; Fay, F.; Scott, C.J.; Abdelghany, S.; Sing, R.R.T.; Garland, M.J.;
Woolfson, A.D. Microneedle-mediated Intradermal nanopartile delivery: Potential for ehnaced
local administration of hydrophobic pre-formed photosensitisers. Photodiagn. Photodynam.
2010, 7, 222–231.
185. Matteucci, M.; Fanetti, M.; Casella, M.; Gramatica, F.; Gavioli, L.; Tormen, M.; Grenci, G.;
Angelis, F.D.; Fabrizio, E.D. Poly vinyl alcohol re-usable masters for microneedles replication.
Microelectron. Eng. 2009, 86, 752–756.
186. Han, M.H.; Kim, D.K.; Kang, S.H.; Yoon, H.R.; Kim, B.Y.; Lee, S.S.; Kim, K.D.; Lee, H.G.
Improvement in antigen-delivery using fabrication of a grooves-embedded microneedle array.
Sens. Actuators B 2009, 137, 274–280.
187. Jin, C.Y.; Han, M.H.; Lee, S.S. Mass producible and biocompatible microneedle patch and
functional verification of its usefulness for transdermal drug delivery. Biomed. Microdevices
2009, 11, 1195–1203.
188. Emam, M.;YH.
A novel microdevice for the treatment of hydrocephalus: design and fabrication of an array of
microvales and microneedles. Microsyst. Technol. 2008, 14, 371–378.
189. Hsu, C.C.; Chen, Y.T.; Tsai, C.H.; Kangl, S.W. Fabrication of Microneedles. In Proceedings of
2nd IEEE International Conference on Nano/Micro Engineered and Molicular System, Bangkok,
Thailand, 16–19 January 2007; pp. 639–642.
190. Kim, Y.C.; Quan, F.S.; Compans, R.W.; Kang, S.M.; Prausnitz, M.R. Formulation and coating of
microneedles with inactivated influenza virus to improve vaccine stability and immunogenicity.
J. Control. Release 2010, 142, 187–195.
Int. J. Mol. Sci. 2011, 12
191. Kato, N.; Kawashima, T.; Shibata, T.; Mineta, T.; Makino, E. Micromachning of a newly
designed AFM probe integrated with hollow microneedle for cellular function analysis.
Microelectr. Eng. 2010, 87, 1185–1189.
192. Hou, W.; Das, B.; Jiang, Y.; Qian, S.; Zheng, X.; Yang, J.; Pi, X.; Liu, H.; Zheng, J.; Zhang, Y.
On A Microfabricated Ti-alloy-based Microneedle Array for Transdermal Drug Delivery,
In Proceedings of 3rd IEEE International Conference on Nano/Micro Engineered and Molecular
System, Sanya, China, 6–9 January 2008; pp. 453–456.
193. Kolli, C.S.; Banga, A.K. Characterization of Solid Maltose Microneedles and their Use for
Transdermal Delivery. Pharm. Res. 2008, 25, 104–113.
Improved piercing of microneedle arrays in
dermatomed human skin by an impact insertion method. J. Control. Release 2008, 128, 80–88.
195. Kim, K.; Lee, J.; High aspect ratio tapered hollow metallic microneedle arrays with microfluidic
interconnector. Microsyst. Technol. 2007, 13, 231–235.
196. Gere, J.; Timoshenko, S. Mechanics of Materials, 4th ed.Upper Saddle River, NJ,
197. Zahn, N.HA.P. Micro fabricated polysilicon microneedles
for minimally invasive biomedical devices. Biomed. Microdevices 2000, 2, 295–303.
198. Aggarwal, P.; Johnston, C.R. Geomertrical effects in mechanical characterizing of microneedle
for biomedical applications. Sens. Actuators B 2004, 102, 226–234.
199. Frick, T.DW.R. Resistance forces acting
on suture needles. J. Biomech. 2001, 34, 1335–1340.
200. Khumpuang, S.; Horade, M.; Fujioka, K.; Sugiyama, S. Geometrical Strengthening and
tip-sharping of a microneedle array fabricated by X-ray lithography. Microsyst. Technol. 2007,
13, 209–214.
201. Davis, S.P.; Martanto, W.; Allen, M.G.; Prausnitz, M.R. Hollow metal microneedles for insulin
delivery to diabetic rats. IEEE Trans. Biomed. Eng. 2005, 52, 909–915.
202. Ashraf, M.W.; Tayyaba, S.; Afzulpurkar, N. MEMS based Polymeric Drug Delivery System.
In Proceedings of 6th IEEE Conference on Automation Science and Engineering (CASE),
Toronto, Canada, 21–24 August 2010; pp. 192–197.
203. Rajaraman, S.; Henderson, H.T. A unique fabrication approach for microneedles using coherent
porous silicon technology. Sens. Actuators B 2005, 105, 443–448.
204. Sammoura, F.; Kang, J.; Heo, Y.; Jung, T.; Lin, L. Polymeric microneedle fabrication using a
microinjection molding technique. Microsyst. Technol. 2007, 13, 517–522.
205. Chen, X.; Kask, A.S.; Crichton, M.L.; McNeilly, C.; Yukiko, S.; Dong, L.; Marshak, J.O.;
Jarrahian, C.; Fernando, G.J.P.; Chen, D.; Koelle, D.M.; Kendall, M.A.F. Improved DNA
vaccination by skin-targeted delivery using dry-coated densely-packed microprojection arrays.
J. Control. Release 2010, 148, 327–333.
206. Milewski, M.; Yerramreddy, T.R.; Ghosh, P.; Crooks, P.A.; Stinchcomb, A.L. In vitro
permeation of a pegylated naltrexone prodrug across microneedle-treated skin. J. Control.
Release 2010, 146, 37–44.
Int. J. Mol. Sci. 2011, 12
207. Khann, P.; Silv, H.; Bhansali, S. Variation in microneedle geometry to increase the shear
strength. Proc. Eng. 2010, 5, 977–980.
208. Bal, S.M.; Kruithof, A.C.; Zwier, R.; Dietz, E.; Bouwstra, J.A.; Lademann, J.; Meinke, M.C.
Influence of microneedle shape on the transport of a fluorescent dye into human skin in vivo.
J. Control. Release 2010, 147, 218–224.
209. Donnelly, R.F.; Garland, M.J.; Morrow, D.I.J.; Migalska, K.; Singh, T.R.R.; Majithiya, R.;
Woolfson, A.D. Optical coherence tomography is a valuable tool in the study of the effects of
microneedle geometry on skin penetration characteristics and in-skin dissolution. J. Control.
Release 2010, 147, 333–341.
210. Crichton, M.L.; Ansaldo, A.; Chen, X.; Prowa, T.W.; Fernando, G.J.P.; Kendall, M.A.F. The
effect of strain rate on the precision of penetration of short densely-packed microprojection array
patches coated with vaccine. Biomaterials 2010, 31, 4562–4572.
211. Pearton, M.; Kang, S.M.; Song, J.M.; Kim, Y.C.; Quan, F.S.; Anstey, A.; Ivory, M.; Prausnitz, M.R.;
Compans, R.W.; Birchall, J.C. Influenza virus-like particles coated onto microneedles can elicit
stimulatory effects on Langerhans cells in human skin. Vaccine 2010, 28, 6104–6113.
212. Li, W.-Z.; Hou, M.-R.; Zhou, J.-P.; Zhou, Y.-Q.; Hao, B.-H.; Liu, T.; Zhang, Y. Super-short
solid silicon microneedles for transdermal drug delivery applications international. J. Pharm.
2010, 389, 122–129.
213. Chu, L.Y.; Prausnitz, M.R. Separable arrowhead microneedles. J. Control. Release 2010
214. Yan, K.; Todo, H.; Sugibayashi, K. Transdermal drug delivery by in-skin electroporation using a
microneedle array. Int. J. Pharm. 2010, 397, 77–83.
215. Yan, G.; Warner, K.S.; Zhang, J.; Sharma, S.; Gale, B.K. Evaluation needle length and density of
microneedle arrays in the pretreatment of skin for transdermal drug delivery. Int. J. Pharm. 2010,
391, 7–12.
216. Song, J.M.; Kim, Y.C.; Barlow, P.G.; Hossain, M.J.; Park, K.M.; Donis, R.O.; Prausnitz, M.R.;
Compans, R.W.; Kang, S.M. Improve protection against avian influenza H5N1 virus by a single
vaccination with virus-like particles in skin using microneedles. Antivir. Res. 2010, 88, 244–247.
217. Zhou, C.P.; Liu, Y.L.; Wang, H.L.; Zhang, P.X.; Zhang, J.L. Transdermal delivery of insulin
using microneedle rollers in vivo. Int. J. Pharm. 2010, 392, 127–133.
218. Quan, F.S.; Kim, Y.C.; Compans, R.W.; Prausnitz, M.R.; Kang, S.M. Dose sparing enabled by
skin immunization with influenza virus-like particle vaccine using microneedles. J. Control.
Release 2010, 147, 326–332.
219. Bussemer, T.; Otto, I.; Bodmeier, R. Pulsatile drug delivery systems. Crit. Rev. Ther. Drug
Carrier Syst. 2001, 18, 433–458.
220. Ma, H.K.; Chen, B.R.; Gao, J.J.; Lin, C.Y. Development of an OAPCP-micropump liquid
cooling system in a laptop. Int. Commun. Heat Mass Transf. 2009, 36, 225–232.
221. Xia, F.; Tadigadapa, S.; Zhang, Q.M. Electroactive polymer based microfluidic pump. Sens.
Actuators A 2006, 125, 346–352.
222. Jeong, O.C.; Konishi, S.; Fabrication and drive test of pneumatic PDMS micro pump. Sens.
Actuators A 2007, 135, 849–856.
Int. J. Mol. Sci. 2011, 12
223. Makino, E.; Shibata, T. Micropump with TiNi shape memory diaphragm actuator. J. Surface
Finish. Soc. Jpn. 2005, 56, 213–218.
224. Ashraf, M. W.; Tayyaba, S.; Nisar, A.; Afzulpurkar, N. MEMS based System for Drug Delivery.
In Proceedings of 6th International Conference on Emerging Technologies (ICET), Islamabad,
Pakistan, 18–19 October, 2010; pp. 82–87.
225. Kabata, A.; Suzuki, H. Microsystem for Injection of Insulin and Monitoring of Glucose
Concentration. In Proceedings of 5th International Conference on Sensors, Irvine, CA, USA,
30 October–3 November 2005; pp. 171–174.
226. Yang, S.C.; Liu, C.H. An Electrolysis-bubble-actuated micropump using electrowetting on
dielectric (EWOD) for 1XN micro-sample switches. In Proceedings of Transducers, Denver,
CO, USA, 21–25 June 2009; pp. 2018–2021.
227. Ma, B.; Liu, S.; Gan, Z.; Liu, G.; Cai, X.; Zhang, H.; Yang, Z. A PZT Insulin Pump Integrated
with a Silicon Micro Needle Array for Transdermal Drug Delivery. In Proceedings of 56th
Electronic Components and Technology Conference, San Diego, CA, USA, 30 May–2 June
2006; pp. 667–681.
228. Tayyaba, S.; Ashraf, M.W.; Afzulpurkar, N. Design and Simulation of Double Lumen Polymeric
Microneedles for Blood Transport. In Proceedings of International Conference on Mechanical
and Electrical Technology (ICMET), Singapore, 10–12 September 2010; pp. 615–618.
229. Najafi, K. Micropackaging technologies for integrated microsystems: Applications to MEMS and
MOEMS. Proc. SPIE 2003, 4979, doi:10.1117/12.484953.
230. 3M. Available online: http://solutions.3m.com/wps/portal/3M/en_WW/DrugDeliverySystems/DDSD
(accessed on 27 April 2011).
231. Birchall, J.C.; Clemo, R.; Anstey, A.; John, D.N. Microneedles in clinical practice-an exploratory
study into the opinions of healthcare professionals and the public. Pharm. Res. 2011, 28, 95–106.
2011 by the authors; licensee MDPI, Basel, Switzerland. This article is an open access article
distributed under the terms and conditions of the Creative Commons Attribution license
Source: http://www.ewp.rpi.edu/hartford/~ernesto/F2014/MPT/MaterialsforStudents/Willer/Ashraf2011-MEMS-Microfluidic-Biomedical.pdf
SPINAL CORD MEDICINEEDUCATIONAL MATERIALS FOR PATIENT AND FAMILY BLADDER MANAGEMENT FOLLOWING SPINAL CORD INJURY/IMPAIRMENT Frazier Rehab Institute DISCLAIMERThe information contained herein is intended to be used in accordance with the treatment plan prescribed by your physician and with the prior approval of your physician. You should not begin using any of the information and/or methods described in these publications until you have consulted your physician. Jewish Hospital & St. Mary's HealthCare, Inc. D.B.A. Frazier Rehab Institute, its affiliates, associates, successors and assigns, as well as its trustees, officers, directors, agents and employees are not liable for any damages resulting from the use of this publication.
Medical data is for informational purposes only. You should always consult your family physician, or one of our referral physicians prior to treatment. It is a phenomena that results when there is an intensification of the disease symptoms and often an expansionof similar symptoms to other places all of a temporary nature,after which the patient is improved or well. Often it appears to someas if they have the flu, and so is described as "the patient having flu-like symptoms." "Flu-like symptoms" is an over-simplification ofwhat happens in varying cases and with varying patients.