Gemstone.umd.edu
TEAM ORGAN
MARCH 27TH, 2009
Sara Moghaddam-Taaheri
1. SPECIFIC AIMS
Though nearly 110,000 people are on the organ transplant waiting list, only 77 people receive
organ transplants daily. The time an organ can remain outside the body plays a crucial role in the organ transplantation system. The current viability of hearts is limited to a mere four to six hours, due to the limitations of the common cold storage method. This limitation influences several key decisions, such as where a heart can be transported to, or where the surgery can be conducted. Current methods of cold static storage have reached their limits in storage time due to the extent of ischemia-reperfusion (I/R) injury induced during static cold storage. The extent of reperfusion injury is directly proportional to preservation time in cold storage, and research has shown that with static storage methods, heart storage time will not exceed six hours. This essentially means that the demands of the organ transplant list will not be met.
Hibernation has been shown to protect against I/R injury. The goal of this proposal is to
investigate a novel preservation method utilizing hydrogen sulfide (H2S) to induce a protective state of
hibernation against I/R injury. A landmark study showed that small mice entered a hibernation-like state when breathing 80 ppm H2S. Researchers have applied the idea of metabolic reduction to the cold
storage of hearts. Hearts stored in 1 uM NaHS added to KH solution for 6 hours showed optimal preservation outcome. The researchers attributed the protection to H2S opening K-ATP channels,
scavenging radical oxidative species (ROS), and possibly lowering metabolism. Researchers have applied the idea of metabolic reduction to the cold storage of hearts.
However, gaseous delivery of H2S is impractical, and all prior research has delivered NaHS either
via machine reperfusion or static storage. Static storage has proven to be much less effective than reperfusion, but machine reperfusion, although a promising approach, is too complex for practical application. Additionally, machine reperfusion induces the production of ROS, which results in I/R injury to the heart. H2S is a strong reducing agent, which eliminates ROS
in vivo. It has also been demonstrated
that these effects are concentration-dependent.
One question that has not been adequately addressed is how long hearts should be exposed to
H2S for optimal protection. Past studies have experimented with either one-time injection of NaHS
solution or 30-minute incubation with H2S before ischemia. H2S as a simple additive to preservation
solution is not expected to remain in the solution for the full duration of preservation. It is estimated that 1/3 of NaHS exists as H2S gas, which can quickly escape from the solution. H2S can also be consumed
by the cell. As such, H2S, if not replenished, may act as a preconditioning stimulus. Some effects of H2S
protection require the continued presence of H2S, such as ROS scavenging during ischemia. Longer
exposure to H2S may also help induce the protective hibernation-like state. We hypothesize that a
continuous release of H2S in the preservation solution will result in better protection of the heart during
cold storage.
Hydrogels are polymer networks that can absorb relatively large amounts of water while
remaining insoluble. Hydrogels exhibit many unique physiochemical properties such as biocompatiblility
and provide a protective haven for many drugs that are normally quickly degraded in circulation. Gelatin is
a natural polymer with inherent biocompatibility, biodegradability, and biologically recognizable moieties,
making it an advantageous polymer for use in drug delivery. It has also been used in cardiac drug
delivery applications with no side effects.
Therefore, our global hypothesis is that controlled delivery
of H2S throughout the heart using gelatin hydrogel microspheres will induce a host of protective
effects, including K-ATP channel opening, ROS scavenging, and inducing an adaptive state of
hibernation that will dramatically prolong heart viability and reduce I/R injury in transplants and
will be easier to implement than machine reperfusion. We plan to develop and investigate a method
of controlled delivery of H2S using hydrogels. The specific aims of the proposed project are:
1. The objective is to evaluate the relationship between concentration of NaHS and its effect on
viability by performing a dose-response test in order to evaluate the concentration of NaHS with
the most beneficial results to tissues. We hypothesize that there is a concentration of NaHS at
which it will no longer be effective as a storage treatment, as it will become toxic and thus reduce
viability of tissue.
Therefore, we propose to investigate the effect of varying NaHS
concentrations on heart cell viability.
2. Hydrogels are biocompatible polymers with a wide variety of applications and parameters. By
controlling the degree of crosslinkage and gelatin acidity, absorption and release rates of NaHS
can be varied until a desired time-release profile of NaHS is achieved.
We propose to
investigate these two biomaterial aspects in the fabrication of gelatin microspheres in
order to determine which factors affect gelatin release of NaHS profile.
3. In order to analyze the clinical applicability of the previously developed concentration profiles,
biological efficacy of the NaHS treatment must be analyzed in comparison with existing methods
of organ storage. Here, we plan to analyze the external validity of our concentration profiles to
clinical application. We hypothesize that controlled delivery of NaHS will improve heart viability
compared to existing methods of storage.
We aim to investigate the effectiveness of our
chosen NaHS release profile compared to existing methods of organ storage, at
prolonging tissue survival during storage using ATP, apoptosis, and creatine kinase
assays. Upon completion of these specific aims, we will have developed a new method for heart
storage that will reduce I/R injury and ROS, but will also be easily applicable to today's organ
transport methods.
2. Background and Significance
2A. Current Organ Storage Methods:
Current methods for organ storage provide a limited time frame that organs can remain viable.
For the human heart, storage time is limited to 4 hours. Additionally, there is an organ donor shortage; as of October 2008, 2,708 patients were registered on the waiting list for a heart transplant. However, there have only been 1,307 donors, all deceased, leaving at least 1,000 still in need ("Donors Recovered in the U.S. by Donor Type," 2008). Since quality organs are limited in supply and always high in demand, prolonging storage time can expand supply by expanding the geographic range of potential donors.
The quality of a donor organ is determined by a variety of factors, including donor age and donor
management prior to procurement of the organ. The duration of hypothermic storage and the perfusion techniques utilized to protect organs from I/R injury (an injury that causes dramatic reprogramming of cell metabolism during organ transplantation) is also extremely important.
I/R injury is a form of injury that may result from organ preservation. Preservation-induced injury
is a major contributing factor to early graft dysfunction in recipients (McLaren & Friend, 2003). I/R injury is damage to the tissue that occurs during reperfusion because oxygen free radicals, which are generated from the byproducts of anaerobic metabolism, cause an acute inflammatory response (McLaren & Friend, 2003).
Static cold storage involves the use of low temperatures and University of Wisconsin (UW)
solution, a commonly used storage solution with its own advantages and disadvantages. The additives of UW solution greatly reduce swelling over previous methods, while hypothermic preservation reduces metabolism and therefore metabolic waste. Additionally, the solution provides rapid cooling and a sterile environment. UW solution lowers aerobic metabolism, but arguably too much; it induces anaerobic metabolism, whose end-products generate oxygen free radicals, leading to I/R injury. Inflammation, tissue damage, and cell death is also prevalent when using UW solution.
Other methods of organ storage are also available, such as the use of perfluorocarbons, which is
an additive that takes up oxygen more efficiently. However, despite nearly two decades of research into potential additives to static cold storage solutions, heart storage time has yet to exceed 8 hours (Skrzypiec-Spring, Grotthus, Szelag, & Schulz, 2007). More existing methods include hypothermic machine perfusion, used mainly for kidneys, and normothermic perfusion, which is performed at 37OC (Jamieson & Friend, 2008). Both are promising, but are limited in their applicability due to the requirement of relatively large pieces of equipment to maintain storage conditions.
Existing techniques for organ storage are inadequate, have limited viability time and high risks of
injury and inflammation. These obstacles create the need for a better means of organ storage.
2B. Hydrogen Sulfide in Organ Preservation Today:
H2S was recently discovered to be an endogenously-produced gaseous signaling molecule
synthesized by the same enzymes that are responsible for L-cysteine metabolism, cystathionine -synthase and cystathionine -lyase (Szabo, 2007a). Other H2S sources include microflora of intestinal
epithelium and the inorganic reduction of elemental sulfur in erythrocytes (Szabo, 2007b). The consumption and degradation of H2S occurs quickly through most mammalian tissues in which it is
produced (Szabo, 2007b). As with the other two endogenously produced gasotransmitters, nitric oxide (NO) and carbon monoxide (CO), H2S enters cells passively through the phospholipid bilayer (Szabo,
2007b). Also like NO and CO, H2S is more commonly known as a toxic gas, and its therapeutic value is
still somewhat controversial (Lefer, 2007b; Szabo, 2007a).
Research has suggested that H2S may play a role in regulating various cardiovascular functions,
including vasodilation, blood pressure, and, in isolated tissues, contraction of smooth muscle (Szabo, 2007b). However, many of H2S's cytoprotective effects may occur only in low concentrations. In situations
of heart disease, H2S levels are lower than in healthy hearts (Szabo, 2007b). Furthermore, micromolar
concentrations of H2S help induce expression of anti-inflammatory or cytoprotective genes. However,H2S
at higher concentrations has pro-inflammatory effects (Szabo, 2007b). H2S also regulates the gene for
subunit V of cytochrome c oxidase, the terminal enzyme complex of the electron transport chain (Blackstone, Morrison, & Roth, 2005). The proposed mechanism through which low concentrations of H2S
reduce metabolic rates is inhibition of cytochrome c oxidase, which blocks oxygen uptake and prevents
ATP production, effectively inducing a suspended-animation-like state in a mouse model (MacDonald & Storey, 1999).
Hibernation, an example of a suspended animation state, can protect organs through
hypometabolism, by which 88% of energy is saved that would otherwise be expended in maintaining euthermic processes (Bian, et al., 2006; Hu, et al., 2007; Ji, et al., 2008; Sivarajah, McDonald, & Thiemermann, 2006; Z. Zhang, Huang, H., Liu, P., Tang, C., & Wang, J., 2007). Hypometabolism is achieved through active controls that coordinate a strong suppression of both ATP-consuming and ATP-generating processes, prioritizing ATP consumption to sustain vital processes (Storey, 1997). In addition, genes controlled by hypoxia-inducible factor alpha (HIF-a) have higher expression levels in hibernation (Storey, 1997). HIF-a helps control a range of cytoprotective responses, including red blood cell proliferation and activation of HO-1 (Bernhardt, et al., 2006).
As hibernation can increase resistance to hypoxic and ischemic threats, the protective effects of
H2S have been investigated in the context of transplantable organs. Studies examining H2S in isolated
hearts confirm several of its cytoprotective effects. Although these studies differ in methodologies and experimental protocols, such as variations in time of ischemia, time of reperfusion, and pre- or post-conditioning, they share several common elements. For example, most studies use a Langendorff perfusion column to perfuse the heart and measure overall function of the heart by monitoring various parameters associated with heart function such as coronary flow, coronary perfusion pressure, heart rate, temperature, and myocardial electrogram. Many studies also use NaHS in solution as an H2S donor to
deliver H2S into the cardiac tissue (Ji, et al., 2008). In addition, research focuses on certain widely
accepted indicators of myocardial injury or recovery, such as left ventricular function, infarct size, creatine kinase markers, ATP content, and apoptosis levels.
Left ventricular function is usually monitored by a latex balloon inserted into the left ventricle,
while cardiac output is measured with a Swan-Ganz catheter placed in the pulmonary artery via the right ventricle. Some studies have shown that NaHS treatments improve left ventricular developed pressure (LVDP) after reperfusion (Hu, et al., 2007; Z. Zhang, Huang, H., Liu, P., Tang, C., & Wang, J., 2007). Other studies have demonstrated the recovery of a greater percentage of LVDP in NaHS-treated hearts (Sivarajah, et al., 2006).
Pretreatment with administration of NaHS, an H2S donor, 15 minutes before myocardial ischemia
has been shown to significantly reduce myocardial infarct size by approximately 26% (Elrod, et al., 2007). A later study confirmed an even greater cytoprotective effect when treated with an H2S donor at the time
of reperfusion, instead of before ischemia, significantly reducing myocardial infarct size by as much as 72%, as revealed by TTC staining (Elrod, et al., 2007). The infarction-reducing effects in this study varied depending on the concentration of H2S; the maximum reduction of 72% was achieved with 50 g/kg of the
H2S donor (Sivarajah, et al., 2006). Other studies also support the idea of concentration-dependent
limitation of infarct size but quote different optimal concentrations. For example, one study found the smallest percentage of infarction with a 1 M concentration of NaHS. In addition, this study also suggests an upper boundary to infarction limitation, as the 100 M concentration of NaHS did not significantly reduce infarction compared to the control (Elrod, et al., 2007).
Studies have also measured the extent of myocyte injury to determine the effects of H2S. One
way to measure myocyte injury is by using myocardial protein markers. Significantly reduced levels of a cardiac-specific isoform of troponin-I in hearts treated with 50 g/kg of the H2S donor confirmed the
protective effect of H2S (Sivarajah, et al., 2006). Measurements showing a concentration-dependent
limitation of the release of creatine kinase, another protein marker, from coronary effluent also indicate the protective role of H2S (Szabo, 2007a). Another method of measuring myocyte injury is through the
trypan blue exclusion assay (Bian, et al., 2006). One study found that the percentage of viable cells increased in a concentration-dependent manner, with the greatest percentage of viable cells seen after ischemia in a solution of 100 M NaHS (Hu, et al., 2007).
ATP content is an important measurement of the protective effects of H2S since it is one of the
best indicators of myocyte recovery (Hu, et al., 2007). Hearts treated with NaHS, an H2S donor, showed
significantly higher levels of myocardial ATP following reperfusion (Hu, et al., 2007). As stated previously, H2S has been proven to lower the metabolic rate (Blackstone, et al., 2005). In return, this lowers the
levels of production of ATP and induces a "hibernation" state in which H2S reduces injury by reducing the
need for high levels of O2.
Isolated hearts treated with an H2S donor also tend to exhibit lower levels of apoptosis. TUNEL
staining identified apoptotic cells with brown nuclear staining (Hu, et al., 2007). Although there is some amount of apoptosis in H2S-treated hearts, it is significantly reduced (Elrod, et al., 2007). In some cases,
H2S-treated hearts were found to have a 59% reduction in the number of apoptotic cells (Hu, et al., 2007;
Z. Zhang, Huang, H., Liu, P., Tang, C., & Wang, J., 2007).
In general, research on H2S in hearts focuses on ATP-activated potassium channels as the main
mechanism of the cytoprotective influence of H2S. Several studies show that the introduction of
glibenclamide, a selective K-ATP channel blocker, blocks the protective effects of H2S (Ji, et al., 2008;
Sivarajah, et al., 2006). More specifically, mitochondrial K-ATP channels were implicated by blockingthese channels with 5-hydroxydecanoate and observing the subsequent blocking of the protective effects of H2S (Jamieson & Friend, 2008).
2C. Methods of Drug Delivery to the Heart:
The safety and efficacy of direct injection into the heart has been investigated in the hopes of
creating a quick and uniform method of drug delivery to the heart. Specifically, researchers have compared intracoronary with intramyocardial injection in terms of efficacy of the delivery of skeletal myoblasts (Fukushima, et al., 2008). Using a 24-gauge catheter over 30 seconds for the injection into the left cardiac vein and a 31-gauge needle for the two intramyocardial injections, researchers found that both methods effectively treated ischemic cardiomyopathy (Fukushima, et al., 2008). In another study, researchers injected mesenchymal stem cells into model rat hearts using a 28-gauge needle at a 45-degree angle (Putnam, 2008).
New areas of study focus on hydrogels as a method of controlled drug delivery (CDD). Hydrogels
are a useful drug delivery system due to their gradual degradation, during which they release a standard amount of product. Studies have shown that these hydrogels can be injected with a 26-gauge needle into the ventricle wall. After injection, compounds can be detected using coloring or labeled tags (Yeo, et al., 2007). Gelatinous hydrogels have been used to promote angiogenesis by delivering basic fibroblast growth factor (bFGF) to the heart. Researchers injected the drug intramuscularly into the left ventricular wall. To assess the potential damage at the injection site, left ventricle function was tested with echocardiography and cardiac catheterization, and comparison with the control groups revealed that the experimental group had a higher, tendency to return toward its original dimensions upon removal of pressure, showing a promising treatment for myocardial infarctions (Sakakibara, et al., 2003). In a related study, researchers injected -cyclodextrin/MPEG-PCL-MPEG hydrogel into the left ventricle of rabbits with induced myocardial infarctions. Echocardiographs and tissue assays revealed that the site of hydrogel injection had increased thickness, decreased stretch and dilation of the ventricle walls, and enhanced viability (Jiang, et al., 2008). This example illustrates that hydrogel compounds and injection procedures are safe and in many cases beneficial for the recipient.
The composition of the hydrogel itself can determine its effectiveness as a drug delivery system.
Many gelatinous hydrogels are composed of lactic or glycolic acid, both of which create an acidic environment that can induce inflammatory response. If the hydrogel is built from a polymer-based system such as polyketal, which degenerates into non-acidic products, the inflammation at the site of injection is minimized.
The site of injection affects the efficacy of the drug delivery as well as the invoked inflammatory
response. Hydrogel microspheres may be injected into the heart vasculature through catheters through three routes: the coronary arteries, the coronary veins, and the coronary sinus. While antegrade injection through the arteries caused a broad, even spread through the tissue with a 73 percent retention, it was associated with microinfarctions. Retrograde injection through the veins and direct injection to the ventricular walls were associated with 22 and 47 percent retention, respectfully. While both approaches avoided lethality, both lacked homogeneity of the spread of molecules and allowed leakage into the lungs. Results suggest that the trans-left ventricular injection site allows more leakage than the trans-coronary sinus (Hoshino, et al., 2006a). Further research evaluated the safety of intracoronary injection into the heart. Researchers found that injecting mesenchymal stem cells into the coronary arteries of dogs caused ischemia, microinfarctions, and ventricular arrhythmias. However, when protein markers and electrocardiagrams were assessed on human patients subjected to injections, no harm was caused, as evidenced by normal levels of troponin, a lack of a systemic inflammatory response (measured by levels of the C-reactive protein), and no arrhythmias (Ben-Dor, Fuchs, & Kornowski, 2006). Other studies have
evaluated the safety of catheter-based injection and ruled out arrhythmias, perforation, embolization, stroke, myocardial inflammation, infection, and increased fibrosis caused by injections. At injection sites in humans, researchers examined tissues and found normal, organized tissue and vascular growth without systemic inflammation. However, five patients did suffer from "angina, pericardial tamponade, high-degree atrioventricular block, ST-segment elevation, myocardial infarction, embolic events, and sepsis" from the injection itself (Ben-Dor, et al., 2006).
Therefore, the literature indicates the overall safety of retrograde and direct injections. Hydrogels
show promise as a controlled drug delivery system, as long as attention is paid to the mode of injection and to the composition of the hydrogel.
2D . Gelatin Microspheres as a Drug Delivery Vehicle
A hydrogel is a network of hydrophilic polymer chains that have a high affinity for water and are
thus able to absorb large quantities, but are prevented from dissolving due to the cross-linked network. Hydrogels tend to be a biocompatible material, and are especially suited to bioengineering applications due to their unique physiochemical properties. Hydrogels have seen applications in plastic surgery, nanotechnology, tissue scaffold engineering, and sustained release delivery systems (Peppas, Hilt, Khademhosseini, & Langer, 2006).
Gelatin hydrogels have been previously used in sustained release systems (Tabata, Hijikata,
Muniruzzaman, & Ikada, 1999). Gelatin hydrogels are particularly well suited to delivery applications because of their enzyme-catalyzed degradation
in vivo; as the drug is released, the gelatin undergoes hydrolysis and degrades into its formulative polypeptide. Hydrogels are also uniquely suited to delivery applications due to their ability to "hide" from the host immune system because of their amino acid composition, thus avoiding the immune system (Dupont, 2002).
There are several release methods available for hydrogels: diffusion-, swelling-, and chemically-
controlled. Diffusion-controlled release rates are largely determined by the mesh size of the polymer network. Swelling-controlled release involves a hydrogel formed with two layers, and upon contact with a liquid, the outer layer swells to form the gel. The release rate is then modulated by the rate of water transport and the thickness of the gel layer. Finally, chemically-controlled release occurs when the degradation of the polymer network is the rate-determining step of the release and diffusion is negligible, or the drug is covalently linked to the hydrogel polymer, and a reaction is necessary to release the drug.
In previous studies using gelatin as a hydrogel, the diffusion-controlled release method was used
to great effect (Hoshino, et al., 2006b; Tabata, et al., 1999). The mesh size of a hydrogel is affected by several factors: polymer molecular weight, polymer composition, and amount of cross-linking; these factors influence the mesh size of the polymer network. Tabata and colleagues (1999) concluded that the gelatin microsphere was a promising carrier of bFGF and went on to use it with promising results for coronary artery disease (Sakakibara, et al., 2003) as well as in a clinical investigation for limb ischemia (Marui, et al., 2007). Although concerns regarding the creation of infarcts with the use of microspheres were once present, it has been demonstrated that gelatin microspheres less than 10 microns in diameter permeate the heart completely, and no infarcts were observed (Hoshino, et al., 2006b).
2E. Alternatives to H2S:
The most widely used method in clinical organ preservation today is cold temperature storage at
4OC (Ahmad, et al., 2004; Zheng, et al., 2008). Improvements to UW solution have come about in the area of impermeants, radical scavengers, and the sodium-potassium gradient. New studies have shown the effectiveness of sucrose (Liu, et al., 1995) as a simpler alternative to impermeants found in UW, as well as trehalose, which confers protection from drying and freezing to membranes in fungi and insects (Hauet & Eugene, 2008). The water-structuring properties of polyethylene glycol (PEG) protect cells from swelling by forming an ice-like barrier. PEG also provides immuno-camouflage by blocking the immunological synapse (Janben, 2003). New radical scavengers that act to inhibit the propagation of ROS include histidine and mannitol (Ramella-Virieux, et al., 1997). Many studies showed that the high potassium (K+)/low sodium (Na+) gradient in UW solution promotes the efflux of intracellular K+, depolarizing the membrane and allowing Ca2+ to enter the cell, which induced vasoconstriction (Liu, et al., 1995). In extracellular-type solutions, flipping the K+/Na+ gradient of the solution has reduced this problem (McAnulty, Reid, Waller, & Murphy, 2002). In addition, a wide range of medicinal additives have been
tested in UW, including apoptosis inhibitors such as pifithrin-a that prevent the transcription of pro-apoptotic proteins like Bax, Ca2+ channel blockers such as nifedipine and verpamil, which control Ca2+influx (Jamieson & Friend, 2008), and trimetazidine, which reduces ATP depletion, scavenges for ROS, and limits Ca2+ influx (t'Hart, et al., 2005).
The purpose of cold storage at 4OC is to reduce metabolic rate and biochemical reactions, to
preserve energy reserves, and to avoid waste buildup. However, low temperature and ischemic conditions limit ATP synthesis, thus limiting vital cellular functions. New techniques have been derived from finding optimal oxygenation and temperature conditions for ATP-synthesis (Jamieson & Friend, 2008). Oxygenation of storage solution helps maintain mitochondrial function for ATP synthesis and anti-oxidant mechanisms. Oxygen persufflation and perfluorchemicals with UW solution (two-layer method) have improved ATP recovery and maintained antioxidants better than cold storage (Matsumoto, et al., 1996). The mild two-layer method, a variation of the two-layer method at 20OC, has led to faster ATP recovery and resuscitation of pancreases. Studies have shown the potential of normothermic preservation, which involves the perfusion of cell-culture media or blood-based perfusate near physiological temperatures, to lengthen storage times and improve graft survival (Stubenitsky, et al., 2000). Another development in the research of additives to cold storage solution is trophic factor supplementation. Adding various growth factors prolonged storage time of kidneys, possibly by accelerating regeneration of tissue or activating protective signaling cascades (Nakao, Kaczorowski, Sugimoto, Billiar, & McCurry, 2008). Together, these new generation methods represent an opposite approach to preservation, namely creating conditions that support rather than inhibit the metabolism of the organ during storage.
One potential organ preservation improvement lies in unlocking cytoprotective genes inherent in
organs. HO-1 is a particularly important gene responsible for breaking down heme (which converts H2O2
into more reactive free radicals) into cytoprotective molecules CO and biliverdin. HO-1 is a heat shock protein that is expressed in various stress situations, such as oxidative stress, hot temperature, ischemic preconditioning, and chemicals (Jamieson & Friend, 2008). The advancement of gene manipulation has made possible the transfer of protective genes to the preserved organ through an engineered virus vector. Successful experiments have transferred various genes including HO-1, Bcl-xl, and Interleukin-10 (Jamieson & Friend, 2008).
It is recognized that improvement of the cold-storage method has met an impasse due to the
inherent problems related to its mechanism. Temperature reduction does indeed lower metabolism by a factor of 10-12, but anaerobic metabolism continues (Jamieson & Friend, 2008; Nakao, et al., 2008). With ischemia: 1) end-products of metabolism such as protons, lactate, and hypoxanthine build-up (Cotter, 2003), 2) ATP levels drop to 50% of normoxic levels within 4 hours of ischemia (Hale, Dai, Dow, & Kloner,2008), 3) impairment of mitochondrial antioxidant defenses occurs, 4) calcium influx and leakage from mitochondria is prevalent. For these reasons, the time in cold storage is proportional to the extent of I/Rinjury. While perfusion techniques appear to attenuate these problems, their use is restricted due to the cost and relative complexity of machinery. Similarly, the setbacks of technology-intensive gene therapy include issues of practicalities making it inadequate for organ preservation.
Using H2S to induce a protective hibernation-like state represents a novel, elegant approach to
the preservation problem. H2S has been shown to reduce metabolism dramatically in mice by reducing
oxygen demands, thereby allowing them to survive lethally anoxic conditions. If H2S can regulate the
reduction of metabolism in organs, then many problems associated with cold storage, such as ATP depletion and ROS formation, may be bypassed.
2F. Donor Management and Heart Extraction Today:
Currently, organ harvesting in the United States comprises many regulatory steps meant to
standardize and maintain the quality of transplantation procedures. Physicians identify potential donors using patient history and immunization records. Before the harvesting can occur, the donor must be identified, declared brain dead, and have given consent. Next, donors undergo tests for HIV, hepatitis B, hepatitis C, Epstein-Barr virus, and the toxoplasma antibody. Negative results must be obtained for each of these tests to ensure that the donor's organs are in healthy condition for transplantation. A blood cell count and blood typing must be performed, followed by a detailed analysis of organ function. This step is also to ensure the viability and quality of organs before they are transplanted into the recipient. To assess the viability of donor organ health for the heart, a standard test includes electrocardiograms. Additionally,
changes in troponin and creatine phosphokinase must be measured. If all tests prove negative, an echocardiogram should be ordered to assess morphology (
Guide to Safety and Quality Assurance for the Transplantation of Organs, Tissues and Cells, 2006).
Removal of the heart is only conducted on brain-dead, heart-beating donors and is carried out as
follows. Donor bodies must be maintained at 90 mmHg systolic blood pressure and a heart rate less than 100 bpm. After a stand-off period has occurred to ensure the death of the donor, surgeons cross-clamp the aorta and perform in situ perfusion through a single cannula. Organs are rapidly cooled because the core body temperature is reduced by the perfusion. En bloc removal of organs and subsequent cold dissection is the superior technique for organ removal because all parts of the organ may be exposed to open air. Also, warm ischemic exposure times are reduced by en bloc removal procedures, contributing to its effectiveness. Moreover, subsequent graft function is significantly improved by a flush with streptokinase (an anticoagulant) at a flow pressure of 150 mmHg; it has been shown to lead to the highest recipient survival rates (Brockmann, Vaidya, Reddy, & Friend, 2006). The heart is then stored in UW solution at a reduced temperature of 4oC (
Guide to Safety and Quality Assurance for the Transplantation of Organs, Tissues and Cells, 2006).
2G. Viability Assessments
I. Cell Viability Assessment: Viability is measured in large part by the viability of the individual cells. Cell
viability is most commonly quantified by staining and analyzing the number of viable cells and the cell
morphology (Bonifacino, Dasso , Harford, Lippincott-Schwartz, & Yamada 2004). There are three main
methodologies used to determine the extent of cell viability: trypan blue stain, propidium iodide stain, and
lactate dehydrogenase (LDH) assay (Gerfen, Holmes, Sibley, Skolnick, & Wray, 2004), each of which has
benefits and limitations. Explant culture systems are first established, where tissues of the heart are
minced into small pieces of about 2-3 mm3 and grown in appropriate medium until subconfluence
(Coligan, et al., 2004). The easiest and most efficient method is the trypan blue dye exclusion test, which
takes three to five minutes (Coligan, et al., 2004). Trypan blue staining operates on the concept that live
cells have intact cell membranes that exclude certain dyes such as trypan blue, eosin, and propidium
(Coligan, et al., 2004). Thus, uptake of the trypan blue dye or alteration to rod-shaped morphology
reveals nonviable cells that cannot exclude the dye (Coligan, et al., 2004; Kabaeva, Zhao, & Michele,
2008). Cells are quantified using a hemacytometer (Coligan, et al., 2004; Kabaeva, et al., 2008). A
limitation of trypan blue is inaccuracy. While some cells are damaged, they may appear viable simply due
to their intact membrane integrity (Coligan, et al., 2004). An alternative method for cell viability
assessment is nuclear staining with propidium iodide, which operates on the same basis as trypan blue
(Coligan, et al., 2004; Kabaeva, et al., 2008; Shivakumar, et al., 2008). Cells are incubated with annexin
V-fluorescein isothiocyanate conjugate, counterstained with propidium iodide, and viewed with
fluorescence microscopy for assessment of cell death (Shivakumar, et al., 2008). Propidium iodide
staining is more accurate but is more time consuming (Coligan, et al., 2004). Lastly, lactate
dehydrogenase (LDH) activity assay assesses cell viability by detecting the amount of LDH released from
cells into the medium, which indicates cell membrane failure (Gerfen, et al., 2004). LDH activity is
determined using spectrophotometry.
II. Heart Viability Assessment: The Langendorff model, also known as the isolated heart reperfusion
model, is the definitive method of heart function assessment. First introduced by Oscar Langendorff in
1897, it has undergone many modifications since and is currently one of the most important techniques
used in the study of heart physiology (Skrzypiec-Spring, et al., 2007). The Langendorff model was
responsible for bringing about an understanding of the roles of temperature, oxygen, and calcium ions for
heart contractile function in the early 1900s, and in recent years, has brought about many important
advances in I/R injury (Clements-Jewery, Hearse, & Curtis, 2002), cell-based therapy (Ohno, et al., 2003),
and especially donor heart preservation (Dobsak, et al., 1999; Ryugo, et al., 2004). The Langendorff
reperfusion model has operated on the same principle since its founding: blood or some other appropriate
perfusate is delivered into the heart through a cannula in the ascending aorta, forcing the leaflets of the
aortic valve closed and therefore forcing the perfusate through the coronary arteries. Once the perfusate
has passed through the coronary arteries, it drains into the right atrium. However, the value of this model
lies in that under the proper temperature, oxygenation, and perfusion fluid conditions, the heart will start
beating again within ten minutes of perfusion (Skrzypiec-Spring, et al., 2007). The Langendorff model
yields highly reproducible results, and its preparations are neither demanding nor time consuming. It
allows for a broad spectrum of parameters, from heart rate to ventricular pressure to cardiac metabolism, to be collected from a single experiment.
3. PRELIMINARY STUDIES
In accordance to the laboratory safety guidelines at the University of Maryland, College Park, we
have been trained by a member of the Department of Environmental Safety in a "New Laboratory Researchers' session." The training demonstrated necessary laboratory procedures, including the safe handling of the hazardous material H2S. In addition to the laboratory safety training, the campus
veterinarian is in the process of training the team in rat heart dissection and tissue handling.
Through preliminary studies, we have investigated different methods of gelatin hydrogel
microspheres fabrication by either the magnetic stir plate or the atomizer. The smallest average diameter of the fabricated microspheres to date is 7 microns. We seek to have an average diameter of microspheres below 10 microns in order to avoid capillary blockage. We are currently exploring the atomizer method, which sprays an aerosol of gelatin particles into gluteraldehyde solution.
There has been much investigation into therapeutic effects of NaHS in solution to protect the
heart from I/R injury. In numerous I/R simulations, NaHS introduced before, during and after ischemia has been shown to reduce infarct size following reperfusion (Bliksoen, Kaljusto, Vaage, & Stenslokken, 2008; Elrod, et al., 2007; Johansen, Ytrehus, & Baxter, 2006). H2S can also attenuate I/R-related cardiac
dysfunction (Bian, et al., 2006). H2S is a strong reducing agent and thus a powerful antioxidant that can
help control ROS (Lowicka & Beltowski, 2007). It is anti-inflammatory and inhibits leukocyte adhesion (Lefer, 2007b). H2S can relax vascular smooth muscle tissue and dilate isolated blood vessels (Lefer,
Investigation into the mechanism by which H2S confers protection has revolved around its action
on K-ATP channels and its inhibitory effect on cytochrome c oxidase, the terminal complex of the electron transport chain. K-ATP channels control calcium influx indirectly by facilitating potassium efflux. H2S, by
opening K-ATP channels, can shorten action potential duration and thereby save energy otherwise used for contraction (Pan, Feng, Lee, Moore, & Bian, 2006; Z. Zhang, Huang, Liu, Tang, & Wang, 2007). Calcium overload can contribute also to the opening of the mitochondrial permeability pore, which dissipates the proton gradient, uncouples ATP synthesis, and leads to apoptosis (Halestrap, Clarke, & Khaliulin, 2007). H2S has been shown to preserve mitochondrial function following ischemia-reperfusion
(Elrod, et al., 2007). Further support of the K-ATP mechanism is the fact that H2S-induced protection can
be abolished by glibenclamide, a K-ATP channel blocker (Johansen, et al., 2006; Z. Zhang, et al., 2007).
Another mechanism by which H2S protects from I/R injury lies in its ability to reduce metabolic
rate. The dramatic hypometabolic effect of H2S on live mammals has been termed "hibernation on
demand" (Roth & Nystul, 2005). Mice breathing 80 ppm H2S had 90% lower metabolic rate, with
reductions in respiratory rate and core body temperature (Blackstone, et al., 2005). Mice breathing 150 ppm entered a suspended animation-like state and were able to survive hypoxia (Blackstone & Roth, 2007). Reduced metabolism lowered ROS generation and preserved ATP content (Elrod, et al., 2007).
Different procedures for delivery of NaHS have been explored. Researchers have introduced
NaHS at different times, for different durations, and at different concentrations. Sulfide preconditioning is a technique whereby NaHS, prior to ischemia, is perfused through the heart at timed intervals separated by perfusion with regular buffer solution. The protection conferred is comparable to the effects of ischemic preconditioning (Pan, et al., 2006). Sulfide preconditioning with 100µM NaHS attenuated I/R-induced arrhythmias and increased mycocyte viability (Bian, et al., 2006). 40µM NaHS introduced at the same time as reperfusion attenuated I/R-induced cardiac dysfunction (Z. Zhang, et al., 2007).
Less research has been invested in NaHS treatment in the context of heart preservation. 1µM
NaHS in Krebs-Heinselet (KH) buffer solution preserved rat hearts in cold storage with results equal in hemodynamic performance and superior in energy preservation to hearts stored in St. Thomas solution (Hu, et al., 2007). Hearts stored with NaHS had higher ATP levels and lower apoptosis index than hearts stored in St. Thomas solution following reperfusion for 30 minutes (Hu, et al., 2007).
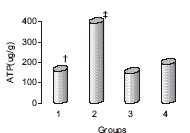
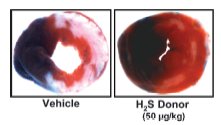
Figure 1. ATP content was better preserved in hearts stored with NaHS added to KH solution (2),
compared with St. Thomas solution (4), after 6 hours of cold storage and 30 minutes of
reperfusion. Group 1 is KH buffer alone, and group 3 is NaHS added to KH solution with
glibenclamide, a K-ATP channel blocker (Hu, et al., 2007).
Figure 2. Representative cross sections of myocardium after 45-min ischemia, 24-hr reperfusion
(with or without H2S donor, NaHS) and TCC staining. H2S reduces infarct size (white area) relative
to the vehicle control.
Figure 3. Relative measurements of infarct size after 30-min ischemia, 120-min reperfusion. NaHS
was added to the perfusate 10 min. before ischemia and administered until 10 min after
reperfusion. H2S at 1 uM NaHS reduces infarct size relative to the vehicle control (no treatment)
(Johansen, et al., 2006).
4. EXPERIMENTAL DESIGN
4A. Overview
Our strategy for organ storage improvement is based upon the hypothesis that H2S can cause a
state of suspended animation in organs, which will reduce the nutrients consumed and the waste produced by the cells, in addition to a wide range of protective effects, including K-ATP channel-openingand ROS scavenging. A novel delivery method for H2S will be developed using hydrogels to extend heart
viability time for transplantation. Specific Aim 1 utilizes a dose-response test to evaluate the relationship between concentration and viability effects of NaHS on myocytes over time. Specific Aim 2 investigates the different release profiles of NaHS by gelatin microsphere by varying the degree of crosslinkage and the pH of the gelatin used. Specific Aim 3 will investigate the effectiveness of the chosen NaHS release profile compared to existing methods of organ storage, at prolonging tissue survival during storage.
4B. Specific Aim 1, Experiment 1
Objective: The objective of this experiment is to determine the rate of H2S metabolism by cardiomyocytes.
With the addition of NaHS to solution, researchers have faced various issues with keeping the concentration of NaHS constant. This may be due to a couple of reasons: (1) the diffusion of H2S into the
atmosphere, (2) the oxidation of sulfide, or (3) the metabolism of H2S by the heart. It is well known that
H2S is produced enzymatically and involved in various physiological reactions of the cardiovascular
system, but whether H2S is significantly metabolized by the cell is unknown (Lefer, 2007a).
Experimental Design: After excision of the heart, tissue will be extracted and myocytes will be isolated for a cell culture, as outlined in the methods section (4H). The cell culture will be split into three experimental groups: (1) Cell culture not exposed to NaHS; (2) Cell culture media (no cells) exposed to 25µM of NaHS; (3) Cell culture exposed to 25µM of NaHS. After 24 hours incubation at 37OC, aqueous H2S levels will be
measured as outlined in the methods section, "Measuring Hydrogen Sulfide in Solution" (4H). 40µMNaHS will be used because it has been shown to have an effect on heart preservation (Bliksoen, et al., 2008). The cell culture media (Group 2) accounts for any NaHS that escapes the media as H2S gas. This
experiment will be conducted in triplicate.
Success Criterion: If there is a statistically significant difference between the H2S levels of the cell cultures
with NaHS and the cell culture media with NaHS, then this experiment will have shown that cardiomyocytes metabolize H2S.
4C. Specific Aim 1, Experiment 2
Objective: The objective of this experiment is to evaluate the relationship between concentration of NaHS
and its effect on heart viability by performing a dose-response test. This relationship determines the most
effective concentration of NaHS to use in later experiments. The hypothesis is that 1µM of NaHS will yield
the most viable cells after cold storage, as previous studies have demonstrated.
Experimental Design: Following excision, rat hearts will be cooled to 4ºC, following standard clinical procedures. A single heart submerged in UW solution will serve as a control (Group 1). In comparison, submersion of a heart in UW solution containing NaHS at concentrations of 1, 25, 50, 75, 100, and 150µM will be tested (Groups 2-6). The left ventricle outer wall will be biopsied and tested for ATP content and apoptosis at 2, 4, 6, and 8 hours. Creatine kinase will be analyzed at the same time points by measuring its presence in the solution. The test will be conducted in triplicate.
Animal requirements: This experiment will require 18 rats (6 groups x Triplicate).
Success Criterion: The success criterion is the evaluation of a concentration-effect relationship of NaHS on myocytes over time. This dose-response test will investigate the concentration that will yield the most viable cells.
4D. Specific Aim 2, Experiment 1
Objective: Previous studies have shown gelatin hydrogels to be an effective drug delivery system for cardiac repair as they provide a biodegradable system that does not require retrieval (Kawai, Suzuki, Tabata, Ikada, & Nishimura, 2000). The absorption and release rates of NaHS in gelatin must be considered in order to achieve desired release profiles of NaHS. Previous studies have demonstrated acidic and basic gelatin to have an effect on the release of the drug due to electrostatic interactions between the gelatin and the molecule (Tabata, et al., 1999). Sorption of NaHS will directly affect the release rate of NaHS over time. The objective of this experiment is to investigate the different time-release profiles of NaHS by varying degrees of gluteraldehyde crosslinking of gelatin with either acidic or basic gelatin. We hypothesize that since increasing concentrations of gluteraldehyde increases crosslinkage, controlling our gluteraldehyde concentration and combining it with a specific gelating type will be an important factor in controlling time release of NaHS. Acidic and basic gelatin have distinct chemical properties. Hence, both will be tested to determine the combination of gluteraldehyde concentration and gelatin acidity that will yield a desirable time-release profile of NaHS.
Experimental Design: Gelatin microspheres of less than 10μm in diameter will be fabricated according to protocol (Tabata, et al., 1999) and will be crosslinked using varying gluteraldehyde (GA) concentrations. We have chosen a 10 micron microsphere diameter to decrease chances of microspheres blocking capillaries upon entering the heart; Hoshino et. al found that 10 micron microspheres were very effective at spreading throughout the entire heart and gave no report of infarcts (Hoshino, et al., 2006b). Separate groups of microspheres will be fabricated with GA concentrations ranging from 1%w/v to 25%w/v. Each different GA %w/v microsphere group will be prepared in duplicates—1 group with acidic gelatin and the other with basic gelatin. These groups will then be loaded with the concentration of NaHS with the highest response and viability as determined from Specific Aim 1.
After fabrication, each set of microspheres will be placed in 25 mL of PBS solution at 4OC, which
mimics current conditions used in clinical settings. Every four hours for a 6-hour period, 2 mL of solution will be extracted to measure H2S released, using the zinc acetate H2S concentration assay for solutions,
described in the methods section (4H, Measuring Hydrogen Sulfide in Solution). The 6-hour time trial correlates with the maximum storage time achieved through machine perfusion (Fitton, et al., 2004). Each experiment will be conducted in triplicate.
Success Criterion: The success criterion of this experiment is to achieve controlled release profiles of NaHS as a result of varying GA concentration and gelatin type, and to select a desired combination of GAconcentration and gelatin type that will give us a desired time-release profile. As the next specific aim will require microspheres with a controlled release rate, the creation of these different release profiles will determine the concentration of GA and the type of gelatin to use.
Preliminary Results: Preliminary hydrogel microspheres fabrication resulted in hydrated microspheres of either one-time injection of NaHS solution or incubation with H2S for 30 min before ischemia less than 10
microns in diameter, as shown in picture below.
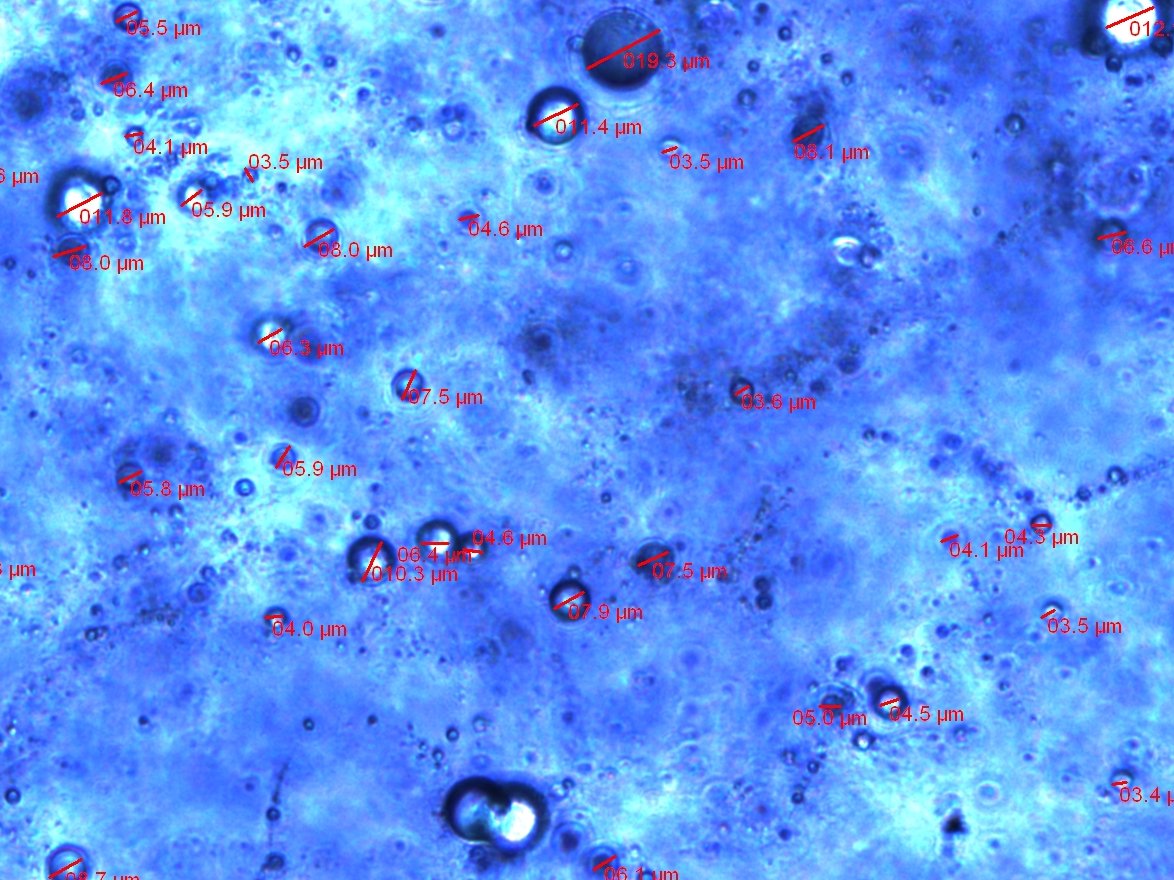
Figure 4. A sample of gelatin microspheres fabricated with the methods specified under 4H and
viewed under an inverted light microscope. The average of the microspheres' diameters is 6.8 ± 4
microns (n=67).
Microsphere Size Distribution
)
7
6
= 15
(n
y
c 10
Figure 5. Size distribution of microspheres fabricated in one batch. N = 67 with an average
diameter of 6.9 ± 4 microns.
NaHS in UW solution
NaHS in UW solution
NaHS in UW solution
NaHS in UW solution
with NaHS loaded
injected with NaHS
injected with PBS
loaded microspheres
loaded microspheres
Figure 6. Experimental groups for Specific Aim 3
4E. Specific Aim 3, Experiment 1
Objective: The objective of this experiment is to evaluate the effects of H2S in UW solution on preserving
the heart. Two experimental groups will be used: (A) heart submerged in UW solution; (B) heart submerged in UW solution with NaHS (see Figure 6). We aim to determine whether H2S has any effects,
such as enhancing or hindering, on the preservative UW solution that is used clinically today. The hypothesis is that H2S will protect the heart by inducing a hibernation-like state in the heart, in addition to
K-ATP channel opening and ROS scavenging, enhancing the UW solution and yielding higher viability of the heart after storage.
Experimental Design: Following excision, rat hearts will be cooled to 4ºC to prevent I/R injury. A single heart submerged in UW solution will serve as a control (A). In comparison, submersion of a heart in UW solution containing NaHS will be tested (B). H2S concentration in the surrounding solution will be
measured using the zinc acetate assay and recorded every 4 hours. At the end of a 6-hour period, the outer wall of each chamber of the heart will be biopsied and the following biological assays will be tested: ATP content, apoptosis, and creatine kinase. Heart function will be assessed using a Langendorff apparatus to determine whether the heart functions satisfactorily.
Success Criterion: The success criterion is to determine the effects of H2S in UW solution on heart
Alternative: As UW solution originally has a protective effect on the heart, it is possible that an added amount of NaHS will not result in a significant difference in heart viability when compared with UW solution by itself. In order to adequately test for any protective or inhibitive effects of H2S, an alternative
approach may be used. In this alternative approach, the heart will be submerged either in (1) KH, which is a buffer solution with no protective effects, or (2) KH with NaHS. Previous studies have indicated that hearts submerged in just KH resulted in poor viability whereas hearts submerged in KH with NaHS resulted in a significant increase in heart viability (Hu et al., 2007).
4F. Specific Aim 3, Experiment 2
Objective: The objective of this experiment is to compare viability effects produced by fixed
concentrations of H2S (produced by static storage, group B) and constant replenishment of H2S
(produced by hydrogel delivery, group C; see Figure 5). We aim to determine how constant exposure to H2S will affect viability of the organ. The hypothesis is that since H2S is depleted very quickly after being
released, K-ATP channel-mediated effects, ROS scavenging, and a constant replenisment of H2S supply
into the heart's surrounding medium will confer more protection by yielding more effective organ hibernation, ROS scavenging, and K-ATP channel opening (Roth & Nystul, 2005).
Experimental Design: Following excision, rat hearts will be arrested and cooled to 4ºC to prevent I/R injury. A single heart submerged in UW solution will serve as a control. Submersion of a heart in UW solution containing NaHS will be compared to hydrogel microsphere delivery. Hydrogel delivery of NaHS will be employed as a mechanism for controlled time-release of H2S. Microspheres will be fabricated as
described in Specific Aim 1 and will contain NaHS concentrations determined to be suitable from Specific Aim 1 conclusions. A single heart will be submerged in UW solution that contains a baseline concentration of NaHS (such as that in the submersion group), and microspheres loaded with NaHS will be placed in medium surrounding the heart. H2S concentration in the surrounding solution will be
monitored and recorded at multiple time points. At the end of our chosen time period, the outer wall of each chamber of the heart will be biopsied and the following biological assays will be tested: ATP content, apoptosis, and creatine kinase. Heart function will be assessed using a Langendorff apparatus to determine whether the heart functions satisfactorily.
Success Criterion: The success criterion is the evaluation of whether constant time-controlled release of NaHS over a given time period yields more viable tissue than static storage of the heart in UW solution containing NaHS.
4G. Specific Aim 3, Experiment 3
Objective: The objective of this experiment is to evaluate potential viability differences between hearts in which the inner chambers have been exposed to H2S first and hearts in which the outer tissues have
been exposed to H2S first. We will be comparing experimental group (C) with (D) (see Figure 6). We aim
to establish whether or not controlled time release of H2S through microsphere injection yields more
viable tissue than controlled time release by means of outer tissue exposure to controlled time release of H2S. We hypothesize that injection of microspheres containing NaHS into the heart will optimize tissue
exposure to H2S, thus maximizing its hibernation-inducing properties.
Experimental Design: Following excision, rat hearts will be cooled and arrested at 4ºC to prevent I/R injury. A single heart submerged in UW solution will serve as a control. Submersion of a heart in UW solution containing NaHS will be compared to hydrogel microsphere delivery. Hydrogel delivery of NaHS will be employed as a mechanism for controlled time-release of H2S. Microspheres will be fabricated as
described in Specific Aim 1 and will contain NaHS concentrations determined to be suitable based on Specific Aim 1 conclusions. A single heart will be submerged in UW solution that contains a baseline concentration of NaHS (such as that in the submersion group), and microspheres loaded with NaHS will be placed in medium surrounding the heart. H2S concentration in the surrounding solution will be
monitored and recorded at multiple time points. In another group, a single heart will be submerged in UW solution that contains a baseline concentration of NaHS, and microspheres loaded with NaHS will be injected into the heart retrograde through the aorta. H2S concentration in surrounding solution will be
monitored and recorded every hour. Another control group will be employed, in which "empty" gelatin microspheres loaded with PBS are injected retrograde through the aorta into the heart. The purpose of this control is to assess the viability effects that are caused by gelatin alone, without regard to NaHS effects. At the end of our chosen time period, the outer wall of each chamber of each heart will be biopsied and the following biological assays will be tested: ATP content, apoptosis, and creatine kinase. Heart function will be assessed using a Langendorff apparatus to determine whether the heart functions satisfactorily.
Success Criterion: The success criterion is an evaluation of whether or not injection of microspheres containing NaHS into the heart yields more viable tissues compared to submersion of the heart into solution containing NaHS-loaded microspheres.
4H. Specific Aim 3, Experiment 4
Objective: The objective of this experiment is to evaluate the effects of gelatin microspheres on the heart.
Two experimental groups will be used: (B) heart submerged in UW solution with NaHS; (E) heart
submerged in UW solution with NaHS and injection of PBS-loaded microspheres (see Figure 6). We aim
to determine whether gelatin microspheres have any effects on the viability of the heart. The hypothesis is
that gelatin microspheres, as a biodegradable system and with a diameter smaller than capillaries, will not
have any significant effects on viability.
Experimental Design: Following excision, rat hearts will be cooled and arrested to 4ºC to prevent I/R injury. A single heart submerged in UW solution with NaHS (B) will be compared to a single heart submerged in UW solution with NaHS and PBS-loaded microspheres (E). H2S concentration in the
surrounding solution will be measured using the zinc acetate assay and recorded at multiple time points. At the end of a 24-hour period, the outer wall of each chamber of the heart will be biopsied and the following biological assays will be tested: ATP content, apoptosis, and creatine kinase. Heart function will be assessed using a Langendorff apparatus to determine whether the heart functions satisfactorily.
Success Criterion: The success criterion is to determine the effects of gelatin microspheres on the heart viability and to validate that injection of microspheres into the interior of the heart will not cause myocardial infarctions or related heart diseases.
4H. Methods
Heart Excision
The rat is prepped for heart excision by heparinizing it with 1-1.25 U/g 15-20 minutes before start of surgery. The rat is then euthanized and the heart is removed. To keep the heart beating, an alternative protocol is necessary – rats will need to be kept under standard conditions: under 12 hours of light and with commercial rat feed and water available ad libitum (Mirtschink, et al., 2008). Following anesthetization by 60mg/kg of sodium pentobarbital and death by decapitation, hearts will be removed within three minutes of euthanization. The chest is opened by the median sternotomy technique (Markel, et al., 2008). The hearts and thoracic organs will be stabilized in order to expose the inferior mesenteric vein and aorta, both of which will be cannulated. The supraceliac abdominal aorta will be encircled. Synchronized aortic cross-clamping will be performed so that the heart can immediately be perfused with KH buffer at 4°C, which provides uninterrupted cooling. The thoracic cavity will be packed with ice (Aydin, et al., 2008). Next, the heart can be removed quickly and immediately immersed in a PBS solution (Quaiserova-Mocko, Novotny, Schaefer, Fink, & Swain, 2008) with the aorta in a vertical direction (Mirtschink et al., 2008). To perfuse the heart, the aorta should be cannulated and the pressure should be around 75 mmHg. The left atria should be resected to insert a latex balloon into the ventricle with a pressure of 5 mmHg. After 15 minutes to allow equilibrium, pacing wires can be attached at 6 Hz, 3V, 2ms or 350 bpm to allow perfusion. (Markel, et al., 2008)
Fabricating Gelatin Microspheres 10 mL of 10 wt% gelatin aqueous solution will be preheated at 45ºC for 1 hour. The solution will be added drop-wise to 350 mL of olive oil while stirring at 1150 rpm for 30 minutes at 4ºC. A modified procedure uses an atomizer to spray fine droplets of gelatin solution into the oil. 100 mL of acetone will be added and stirred for 1 hour. The microspheres that form will be washed once with acetone and centrifuged at 4000 rpm for 5 minutes and then washed once with isopropyl alcohol and centrifuged at 4000 rpm for 5 minutes. The microspheres will be collected by centrifugation at 5000 rpm for 5 min at 4ºC. They will then be suspended in isopropyl alcohol. Microspheres will be put through a sieve with an aperture of 32 µm. Microspheres will be centrifuged again and air dried at 4ºC. Microspheres will be placed in a 2 wt% glutaraldehyde solution containing 0.1 wt% Tween 80 for 12 hours at 4ºC. Microspheres will be placed in 100 mL of 10 mM glycine solution containing 0.1 wt% Tween 80 at 37ºC for 30 min. Microspheres will be washed twice with the 0.1 wt% Tween 80-solution and centrifuged at 4000 rpm for 5 min at 4ºC. The spheres will be freeze dried for 3 days. Different concentrations of aqueous H2S will be added to 2 mg of
the gelatin microspheres and will be left in room temperature for 30 min. (Kawai et al., 1999)
Hydrogen Sulfide Delivery (antegrade injection)Various concentrations of NaHS will be injected antegrade through the coronary artery with a catheter. A 3.5 mm over-the-wire balloon catheter (Boston Scientific Corporation, MA) will be used and guided to the just-distal site of the first diagonal branch with a guiding catheter. Once the wire balloon catheter is in place, the guiding wire will be removed. The balloon will then be inflated to two atm. The microspheres that were previously prepared will be diluted in 5 mL of saline and injected through the wire lumen of the balloon catheter. (Hoshino et al., 2006)
Heart Tissue IsolationFollowing excision of the heart, both the right ventricle and left ventricle (LV) free walls will be rapidly removed and cut into apical, mid and basal portions. The middle regions of these strips will be discarded. The apical and basal regions from the LV free wall will be further divided into epicardial and endocardial layers as follows. The LV apical and basal tissue strips will be pinned to a polymer-based surface with the epicardial surface facing upwards. A thin epicardial layer, approximately 1.5¨C2 mm in thickness (visual inspection), will be dissected and retained. The underlying mid-myocardial layer will be removed and discarded. The remaining endocardial layer will be retained. All tissue strips will be rapidly frozen in liquid nitrogen. For each set of RNA isolation the dissected tissue strips from 3 hearts will be pooled. (Teutsch, et al., 2007)
Cardiac Myocyte IsolationCardiac tissue will be isolated with scissors, minced, and treated with collagenase. Cells will be collected through centrifugation, plated, and incubated for 1.5 hours. The supernatant will be collected a second time. Cells will be plated on a collagen-coated dish at an appropriate density with Dulbecco-modified
Eagle Medium containing 10% FBS, 1% penicillin/streptomycin, and .1 mM bromodeoxyuridine (BrdU), and incubated for 24 hours in a humidified chamber at 37°C and 5% CO2. Medium will be changed to
contraction medium (Modified Eagle Medium with 10% calf serum, 1% penicillin/streptomycin, and .1 mM BrdU. Cells will be incubated again under the same conditions. (Harada & Isomura, 2006)
Measuring Hydrogen Sulfide using High-Performance Liquid Chromatography Tissues samples weighing 50-150 mg will be excised from the frozen samples and put in a clear glass crimp-top vial with molded conical bottom (Sun International, Wilmington, NC). Vials will be sealed using a Teflon septum and for every mg of sample, 1 µL of tetraethylammonium hydroxide (TEAH, 35% aqueous solution, SACHEM, Austin, TX) will be added via gastight syringe (Hamilton, Reno, NV). The vials will be centrifuged for 5 min at 3000 x g at 4ºC. The vials will be left in room temperature for 24 hours and then for every mg of sample, 8 µL of 28 mM NaOH will be added to the vial. The vials will be centrifuged for 5 min at 3000 x g. 100 µL of the supernatant will be added to 400 µL of 28 mM NaOH in a polypropylene ConSert vial (Sun International, Wilmington, NC). The sample will then be injected into the liquid chromatography system and the components will be separated using high-performance liquid chromatography (HPLC). Sulfide will be detected using an electrochemical detector equipped with a guard cell and an analytical cell with silver target. (Dorman et al., 2002)
Measuring Hydrogen Sulfide in Solution500 µL solution containing an unknown amount of H2S will be added to an Eppendorf tube already
containing zinc acetate (1% w/v, 250 µL) to trap H2S. Then, 133 µL of 20 µM N,N-dimethyl-p-
phenylenediamine sulphate in 7.2 M HCl will be added, followed by 133 µL 30µM FeCl3 in 1.2 M HCl.
Absorbance will then be analyzed at 670 nm using a microplate spectrophotometer. (Pan, et al., 2006)
ATP AssayThe ATP assay will be conducted following manufacturer's protocol. The Bioluminescence Assay from Biovision Inc. will be used.
Apoptosis AssayTo measure cell apoptosis levels, the TUNEL assay will be used as first described by Gavrieli et al. (1992). For tissue preparation, tissue samples will first be embedded in formaldehyde and paraffin, and then deparaffinized and hydrated in different concentrations of ethanol solution. To prepare the cardiomyocytes, the cardiac tissue will be minced and the cells will be separated using a metal pins comb. The cell suspension will then be filtered through metal mesh and centrifuged to concentrate the cells in the pellet. Then, dexamethasone will be added and the cells incubated for up to 6 hours. After incubation, DNA nick end labeling will be performed on the tissue sections as well as the cardiomyocytes. Then the samples will be treated with DNAase in situ and the DNA extracted. To see the results, the DNA samples will be separated using agarose gel electrophoresis. (Gavrieli, Sherman, & Bensasson, 1992)
Creatine Kinase AssayDetection of creatine kinase cardiac isoenzyme (CK-MB) can be performed using a commercial kit, CK-MB Granutest (Merck, Darmstadt, Germany). A sample will be obtained from the storage solution at given timepoints and analyzed using manufacturer's protocol.
5. Proposed Budget
Summer 2009 Fall 2009
Dates of Period
Months in Period
Equipment Over $5,000
Total Equipment Over $5,000
Equipment Under $5,000
Animal Purchase
ATP Assay Kit
Creatine Kinase Assay Kit
Apoptosis Assay Kit
Hydrogel Raw Materials
Total Equipment Under $5,000
Total Project Costs
6. TIMELINE
We will begin our research in the spring of 2009 by presenting our thesis and applying for outside
grants. We will also begin with Experiments 1-1 (section 4B) and 1-2 (4C). During the summer of 2009, we will begin our study of hydrogel cross linkage by completing Experiment 2-1 (4D), which assesses the controlled release of hydrogels in cardiac tissue in order to obtain quickly uniform distribution. We will also continue to locate grants. During fall 2009 and winter 2010, we will complete Experiments 3-1 and 3-2, respectively. We will develop an outline of our final thesis and identify drug delivery experts. During the spring of 2010, we will complete experiments 3-3 and 3-4. During the summer of 2010, we will continue data analysis and apply it to our draft of our final thesis. During the fall of 2010, we will complete a final draft of our thesis, to be presented at the Team Thesis Conference in the spring of 2011.
Thesis Presentation
7. VERTEBRATE ANIMALS
All procedures being used with live rats have been previously approved by IACUC.
All rats used will be skeletally mature, Sprague-Dawley adult male rats, weighing approximately 220-270 grams.
The proposal calls for 34 hearts. See table 6 for specific justification.
Specific Aim
Number of subjects
Specific Aim 1, Experiment 1
Specific Aim 1, Experiment 2
Specific Aim 2, Experiment 1
Specific Aim 3, Experiment 1 to 4
Table 6: Detailed listing of proposed number of animal subjects required
All euthanization and care procedures will be carried out by staff of the Tissue Engineering Laboratory, of the Bioengineering Department, Clark School of Engineering, University of Maryland. All students have been trained by the Occupational Safety and Health lab safety training program.
Rats will be used as a source of hearts to model the cardioprotective effects of NaHS (Specific Aims 1 & 3).
Assurance of Minimal Discomfort: All live animal manipulations will be performed under veterinary
supervision in the approved animal facilities at the University of Maryland.
All animals will be euthanized using a carbon dioxide inhalation under previously IACUC approved protocols.
8. LITERATURE CITED
Ahmad, N., Hostert, L., Pratt, J. R., Billar, K. J., Potts, D. J., & Lodge, J. P. (2004). A pathophysiologic
study of the kidney tubule to optimize organ preservation solutions. Kidney Int, 66(1), 77-90.
Aydin, U., Yazici, P., Kazimi, C., Bozoklar, A., Sozbilen, M., Zeytunlu, M., et al. (2008). Simultaneous air
transportation of the harvested heart and visceral organs for transplantation. Transplant Proc, 40(1), 44-46.
Ben-Dor, I., Fuchs, S., & Kornowski, R. (2006). Potential hazards and technical considerations associated
with myocardial cell transplantation protocols for ischemic myocardial syndrome. J Am Coll Cardiol, 48(8), 1519-1526.
Bernhardt, W. M., Campean, V., Kany, S., Jurgensen, J. S., Weidemann, A., Warnecke, C., et al. (2006).
Preconditional activation of hypoxia-inducible factors ameliorates ischemic acute renal failure. J Am Soc Nephrol, 17(7), 1970-1978.
Bian, J. S., Yong, Q. C., Pan, T. T., Feng, Z. N., Ali, M. Y., Zhou, S., et al. (2006). Role of hydrogen
sulfide in the cardioprotection caused by ischemic preconditioning in the rat heart and cardiac myocytes. J Pharmacol Exp Ther, 316(2), 670-678.
Blackstone, E., Morrison, M., & Roth, M. B. (2005). H2S induces a suspended animation-like state in
mice. Science, 308(5721), 518.
Blackstone, E., & Roth, M. B. (2007). Suspended animation-like state protects mice from lethal hypoxia.
Shock, 27(4), 370-372.
Bliksoen, M., Kaljusto, M. L., Vaage, J., & Stenslokken, K. O. (2008). Effects of hydrogen sulphide on
ischaemia-reperfusion injury and ischaemic preconditioning in the isolated, perfused rat heart. Eur J Cardiothorac Surg, 34(2), 344-349.
Bonifacino, J. S., Dasso , M., Harford, J. B., Lippincott-Schwartz, J., & Yamada , K. M. (Eds.). (2004).
Current Protocols in Cell Biology: John Wiley & Sons, Inc.
Brockmann, J. G., Vaidya, A., Reddy, S., & Friend, P. J. (2006). Retrieval of abdominal organs for
transplantation. Br J Surg, 93(2), 133-146.
Clements-Jewery, H., Hearse, D. J., & Curtis, M. J. (2002). Independent contribution of catecholamines to
arrhythmogenesis during evolving infarction in the isolated rat heart. Br J Pharmacol, 135(3), 807-815.
Coligan, J. E., Bierer, N., Kruisbeek, A. M., Margulies, D. H., Shevach, E. M., & Strober, W. (Eds.).
(2004). Current Protocols in Immunology: John Wiley & Sons, Inc.
Cotter, P. Z. (2003). Evaluation of an oxygen delivering mobile perfusion device for organ transport.
Texas Tech University.
Dobsak, P., Courderot-Masuyer, C., Zeller, M., Vergely, C., Laubriet, A., Assem, M., et al. (1999).
Antioxidative properties of pyruvate and protection of the ischemic rat heart during cardioplegia. J Cardiovasc Pharmacol, 34(5), 651-659.
Donors Recovered in the U.S. by Donor Type (2008). Retrieved October 11, 2008, from
Dupont, A. L. (2002). Study of the degradation of gelatin in paper upon aging using aqueous size-
exclusion chromatography. J Chromatogr A, 950(1-2), 113-124.
Elrod, J. W., Calvert, J. W., Morrison, J., Doeller, J. E., Kraus, D. W., Tao, L., et al. (2007). Hydrogen
sulfide attenuates myocardial ischemia-reperfusion injury by preservation of mitochondrial function. Proc Natl Acad Sci U S A, 104(39), 15560-15565.
Fitton, T. P., Wei, C., Lin, R., Bethea, B. T., Barreiro, C. J., Amado, L., et al. (2004). Impact of 24 h
continuous hypothermic perfusion on heart preservation by assessment of oxidative stress. Clin Transplant, 18 Suppl 12, 22-27.
Fukushima, S., Coppen, S. R., Lee, J., Yamahara, K., Felkin, L. E., Terracciano, C. M., et al. (2008).
Choice of cell-delivery route for skeletal myoblast transplantation for treating post-infarction chronic heart failure in rat. PLoS ONE, 3(8), e3071.
Gavrieli, Y., Sherman, Y., & Bensasson, S. A. (1992). Identification of Programmed Cell-Death Insitu Via
Specific Labeling of Nuclear-DNA Fragmentation. Journal of Cell Biology, 119(3), 493-501.
Gerfen, C. R., Holmes, A., Sibley, D., Skolnick, P., & Wray, S. (Eds.). (2004). Current Protocols in
Neuroscience: John Wiley & Sons, Inc.
Guide to Safety and Quality Assurance for the Transplantation of Organs, Tissues and Cells (2006). (3rd
ed.). Strasbourg: Council of Europe Publishing.
Hale, S. L., Dai, W., Dow, J. S., & Kloner, R. A. (2008). Mesenchymal stem cell administration at coronary
artery reperfusion in the rat by two delivery routes: a quantitative assessment. Life Sci, 83(13-14), 511-515.
Halestrap, A. P., Clarke, S. J., & Khaliulin, I. (2007). The role of mitochondria in protection of the heart by
preconditioning. Biochim Biophys Acta, 1767(8), 1007-1031.
Harada, T., & Isomura, A. (2006). Spontaneous formation of cell clusters in a cardiac cell culture system.
Progress of Theoretical Physics Supplement(161), 107-118.
Hauet, T., & Eugene, M. (2008). A new approach in organ preservation: potential role of new polymers.
Kidney Int, 74(8), 998-1003.
Hoshino, K., Kimura, T., De Grand, A. M., Yoneyama, R., Kawase, Y., Houser, S., et al. (2006a). Three
catheter-based strategies for cardiac delivery of therapeutic gelatin microspheres. Gene Ther, 13(18), 1320-1327.
Hoshino, K., Kimura, T., De Grand, A. M., Yoneyama, R., Kawase, Y., Houser, S., et al. (2006b). Three
catheter-based strategies for cardiac delivery of therapeutic gelatin microspheres. Gene therapy, 13(18), 1320-1327.
Hu, X., Li, T., Bi, S., Jin, Z., Zhou, G., Bai, C., et al. (2007). Possible role of hydrogen sulfide on the
preservation of donor rat hearts. Transplant Proc, 39(10), 3024-3029.
Jamieson, R. W., & Friend, P. J. (2008). Organ reperfusion and preservation. Front Biosci, 13, 221-235.
Janben, H., Janben, P., & Broelsch, C. (2003). Celsior solution compared with University of Wisconsin
solution (UW) and histidinetryptophan-ketoglutarate solution (HTK) in the protection of human hepatocytes against ischemia-reperfusion in jury. Transplantation International, 16, 515-522.
Ji, Y., Pang, Q. F., Xu, G., Wang, L., Wang, J. K., & Zeng, Y. M. (2008). Exogenous hydrogen sulfide
postconditioning protects isolated rat hearts against ischemia-reperfusion injury. Eur J Pharmacol, 587(1-3), 1-7.
Jiang, X. J., Wang, T., Li, X. Y., Wu, D. Q., Zheng, Z. B., Zhang, J. F., et al. (2008). Injection of a novel
synthetic hydrogel preserves left ventricle function after myocardial infarction. J Biomed Mater Res A.
Johansen, D., Ytrehus, K., & Baxter, G. F. (2006). Exogenous hydrogen sulfide (H2S) protects against
regional myocardial ischemia-reperfusion injury--Evidence for a role of K ATP channels. Basic Res Cardiol, 101(1), 53-60.
Kabaeva, Z., Zhao, M., & Michele, D. E. (2008). Blebbistatin extends culture life of adult mouse cardiac
myocytes and allows efficient and stable transgene expression. Am J Physiol Heart Circ Physiol, 294(4), H1667-1674.
Kawai, K., Suzuki, S., Tabata, Y., Ikada, Y., & Nishimura, Y. (2000). Accelerated tissue regeneration
through incorporation of basic fibroblast growth factor-impregnated gelatin microspheres into artificial dermis. Biomaterials, 21(5), 489-499.
Lefer, D. J. (2007a). A new gaseous signaling molecule emerges: cardioprotective role of hydrogen
sulfide. Proceedings of the National Academy of Sciences of the United States of America, 104(46), 17907-17908.
Lefer, D. J. (2007b). A new gaseous signaling molecule emerges: cardioprotective role of hydrogen
sulfide. Proc Natl Acad Sci U S A, 104(46), 17907-17908.
Liu, C. J., Bando, T., Hirai, T., Hirata, T., Yagi, K., Yokomise, H., et al. (1995). Improved 20-hour canine
lung preservation with a new solution--ET-Kyoto solution. Eur J Cardiothorac Surg, 9(10), 548-552.
Lowicka, E., & Beltowski, J. (2007). Hydrogen sulfide (H2S) - the third gas of interest for pharmacologists.
Pharmacol Rep, 59(1), 4-24.
MacDonald, J. A., & Storey, K. B. (1999). Regulation of ground squirrel Na+K+-ATPase activity by
reversible phosphorylation during hibernation. Biochem Biophys Res Commun, 254(2), 424-429.
Markel, T. A., Wang, Y., Herrmann, J. L., Crisostomo, P. R., Wang, M., Novotny, N. M., et al. (2008).
VEGF Is Critical for Stem Cell Mediated Cardioprotection and a Crucial Paracrine Factor for Defining the Age Threshold in Adult and Neonatal Stem Cell Function. Am J Physiol Heart Circ Physiol.
Marui, A., Tabata, Y., Kojima, S., Yamamoto, M., Tambara, K., Nishina, T., et al. (2007). A novel
approach to therapeutic angiogenesis for patients with critical limb ischemia by sustained release of basic fibroblast growth factor using biodegradable gelatin hydrogel: an initial report of the phase I-IIa study. Circ J, 71(8), 1181-1186.
Matsumoto, S., Kuroda, Y., Fujita, H., Tanioka, Y., Kim, Y., Sakai, T., et al. (1996). Resuscitation of
ischemically damaged pancreas by the two-layer (University of Wisconsin solution/perfluorochemical) mild hypothermic storage method. World J Surg, 20(8), 1030-1034.
McAnulty, J. F., Reid, T. W., Waller, K. R., & Murphy, C. J. (2002). Successful six-day kidney preservation
using trophic factor supplemented media and simple cold storage. Am J Transplant, 2(8), 712-718.
McLaren, A. J., & Friend, P. J. (2003). Trends in organ preservation. Transpl Int, 16(10), 701-708.
Mirtschink, P., Stehr, S. N., Pietzsch, H. J., Bergmann, R., Pietzsch, J., Wunderlich, G., et al. (2008).
Modified "4 + 1" mixed ligand technetium-labeled fatty acids for myocardial imaging: evaluation of myocardial uptake and biodistribution. Bioconjug Chem, 19(1), 97-108.
Nakao, A., Kaczorowski, D. J., Sugimoto, R., Billiar, T. R., & McCurry, K. R. (2008). Application of heme
oxygenase-1, carbon monoxide and biliverdin for the prevention of intestinal ischemia/reperfusion injury. J Clin Biochem Nutr, 42(2), 78-88.
Ohno, N., Fedak, P. W., Weisel, R. D., Mickle, D. A., Fujii, T., & Li, R. K. (2003). Transplantation of
cryopreserved muscle cells in dilated cardiomyopathy: effects on left ventricular geometry and function. J Thorac Cardiovasc Surg, 126(5), 1537-1548.
Pan, T. T., Feng, Z. N., Lee, S. W., Moore, P. K., & Bian, J. S. (2006). Endogenous hydrogen sulfide
contributes to the cardioprotection by metabolic inhibition preconditioning in the rat ventricular myocytes. J Mol Cell Cardiol, 40(1), 119-130.
Peppas, N. A., Hilt, J. Z., Khademhosseini, A., & Langer, R. (2006). Hydrogels in biology and medicine:
From molecular principles to bionanotechnology. Advanced Materials, 18(11), 1345-1360.
Putnam, D. (2008). Drug delivery: the heart of the matter. Nat Mater, 7(11), 836-837.
Quaiserova-Mocko, V., Novotny, M., Schaefer, L. S., Fink, G. D., & Swain, G. M. (2008). CE coupled with
amperometric detection using a boron-doped diamond microelectrode: validation of a method for endogenous norepinephrine analysis in tissue. Electrophoresis, 29(2), 441-447.
Ramella-Virieux, S. G., Steghens, J. P., Barbieux, A., Zech, P., Pozet, N., & Hadj-Aissa, A. (1997).
Nifedipine improves recovery function of kidneys preserved in a high-sodium, low-potassium cold-storage solution: study with the isolated perfused rat kidney technique. Nephrol Dial Transplant, 12(3), 449-455.
Roth, M. B., & Nystul, T. (2005). Buying time in suspended animation. Sci Am, 292(6), 48-55.
Ryugo, M., Sawa, Y., Ono, M., Fukushima, N., Aleshin, A. N., Mizuno, S., et al. (2004). Myocardial
protective effect of human recombinant hepatocyte growth factor for prolonged heart graft preservation in rats. Transplantation, 78(8), 1153-1158.
Sakakibara, Y., Tambara, K., Sakaguchi, G., Lu, F., Yamamoto, M., Nishimura, K., et al. (2003). Toward
surgical angiogenesis using slow-released basic fibroblast growth factor. Eur J Cardiothorac Surg, 24(1), 105-111; discussion 112.
Shivakumar, K., Sollott, S. J., Sangeetha, M., Sapna, S., Ziman, B., Wang, S., et al. (2008). Paracrine
effects of hypoxic fibroblast-derived factors on the MPT-ROS threshold and viability of adult rat cardiac myocytes. Am J Physiol Heart Circ Physiol, 294(6), H2653-2658.
Sivarajah, A., McDonald, M. C., & Thiemermann, C. (2006). The production of hydrogen sulfide limits
myocardial ischemia and reperfusion injury and contributes to the cardioprotective effects of preconditioning with endotoxin, but not ischemia in the rat. Shock, 26(2), 154-161.
Skrzypiec-Spring, M., Grotthus, B., Szelag, A., & Schulz, R. (2007). Isolated heart perfusion according to
Langendorff---still viable in the new millennium. J Pharmacol Toxicol Methods, 55(2), 113-126.
Storey, K. B. (1997). Metabolic regulation in mammalian hibernation: enzyme and protein adaptations.
Comp Biochem Physiol A Physiol, 118(4), 1115-1124.
Stubenitsky, B. M., Booster, M. H., Brasile, L., Araneda, D., Haisch, C. E., & Kootstra, G. (2000).
Exsanguinous metabolic support perfusion--a new strategy to improve graft function after kidney transplantation. Transplantation, 70(8), 1254-1258.
Szabo, C. (2007a). Hydrogen sulfide and its therapeutic potential. Nature Reviews Drug Discovery(6),
Szabo, C. (2007b). Hydrogen sulphide and its therapeutic potential. Nature Reviews. Drug Discovery,
t'Hart, N. A., van der Plaats, A., Faber, A., Leuvenink, H. G. D., Olinga, P., Wiersema-Buist, J., et al.
(2005). Oxygenation during hypothermic rat liver preservation: an in vitro slice study to demonstrate beneficial or toxic oxygenation effects. Liver Transplantation, 11(11), 1403-1411.
Tabata, Y., Hijikata, S., Muniruzzaman, M., & Ikada, Y. (1999). Neovascularization effect of
biodegradable gelatin microspheres incorporating basic fibroblast growth factor. Journal of biomaterials science, 10(1), 79-94.
Teutsch, C., Kondo, R. P., Dederko, D. A., Chrast, J., Chien, K. R., & Giles, W. R. (2007). Spatial
distributions of Kv4 channels and KChip2 isoforms in the murine heart based on laser capture microdissection. Cardiovasc Res, 73(4), 739-749.
Yeo, Y., Geng, W., Ito, T., Kohane, D. S., Burdick, J. A., & Radisic, M. (2007). Photocrosslinkable
hydrogel for myocyte cell culture and injection. J Biomed Mater Res B Appl Biomater, 81(2), 312-322.
Zhang, Z., Huang, H., Liu, P., Tang, C., & Wang, J. (2007). Hydrogen sulfide contributes to
cardioprotection during ischemia-reperfusion injury by opening K ATP channels. Can J Physiol Pharmacol, 85(12), 1248-1253.
Zhang, Z., Huang, H., Liu, P., Tang, C., & Wang, J. (2007). Hydrogen sulfide contributes to cardioprotection during ischemia-reperfusion injury by opening KATP channels. Canadian Journal of Physiology and Pharmacology.(85), 1248-1253.
Zheng, J. H., Min, Z. L., Li, Y. L., Zhu, Y. H., Ye, T. J., Li, J. Q., et al. (2008). A modified CZ-1 preserving
solution for organ transplantation: comparative study with UW preserving solution. Chin Med J (Engl), 121(10), 904-909.
Source: http://www.gemstone.umd.edu/team-sites/classof2011/organ/files/proposal_033109.pdf
GENERICOS CENTROAMERICANOS, S.A. AÑO IX No. 68 Actualidad MédicaEspecial para Folia Médica: Dr Carlos FernándezEsteroides en el tratamiento inicial de la SepsisEl dolor lumbar: Dr E. J. MerladetFibra y cáncer colorrectalOzonoterapia¿Cuándo mata la enfermedad coronaria?más.
Abstracts presented at the "Students Scientific Day" at Faculty of Dentistry April 4-4-2015 Prepared by: Students of the Faculty of Dentistry at The University of Jordan Edited by: Dr Najla a Kasabrah Dr Yazan Hassona Pigminted Lesion On The Alveolar Ridge