Mpie.de
M.I. Muglali, A. Erbe, Y. Chen, C. Barth, P. Koelsch, M. Rohwerder:
Electrochimica Acta,
90, 17-
26 (
2013).
Final copy-edited version of the manuscript is available from:
Modulation of electrochemical hydrogen evolution rate
by araliphatic thiol monolayers on gold
Mutlu I. Muglalia, Andreas Erbea,∗, Ying Chena,b, Christoph Barthc,
Patrick Koelschc,d, Michael Rohwerdera,1,∗∗
a
Max-Planck-Institut f¨ur Eisenforschung GmbH, Department of Interface Chemistry and
Surface Engineering, Max-Planck-Str. 1, 40237 D¨
b
Center for Electrochemical Sciences, Ruhr-Universit¨at Bochum, Universit¨atstr. 150,
44780 Bochum, Germany
c
Institute of Toxicology and Genetics, Karlsruhe Institute of Technology, Postfach 3640,
76021 Karlsruhe, Germany
d
National ESCA and Surface Analysis Center for Biomedical Problems, Department of
Bioengineering, University of Washington, Box 35170, Seattle, Washington 98195-1750,
Electroreductive desorption of a highly ordered self-assembled monolayer
(SAM) formed by the araliphatic thiol (4-(4-(4-pyridyl)phenyl)phenyl)meth-
anethiol leads to a concurrent rapid hydrogen evolution reaction (HER).
The desorption process and resulting interfacial structure were investigated
by voltammetric techniques,
in situ spectroscopic ellipsometry, and
in situ
vibrational sum–frequency–generation (SFG) spectroscopy. Voltammetric
experiments on SAM-modified electrodes exhibit extraordinarily high peak
currents, which differ between Au(111) and polycrystalline Au substrates.
Association of reductive desorption with HER is shown to be the origin of
∗Corresponding author; Phone: +49 211 6792 890, Fax: +49 211 6792 218
∗∗Corresponding author; Phone: +49 211 6792 914, Fax: +49 211 6792 218
Email addresses: [email protected],
[email protected] (Andreas Erbe),
[email protected] (Michael Rohwerder)
1 ISE member.
Preprint submitted to Electrochimica Acta
November 24, 2012
the observed excess cathodic charges. The studied SAM preserves its two–
dimensional order near Au surface throughout a fast voltammetric scan even
when the vertex potential is set several hundred millivolt beyond the des-
orption potential. A model is developed for the explanation of the observed
rapid HER involving ordering and pre–orientation of water present in the
nanometer–sized reaction volume between desorbed SAM and the Au elec-
trode, by the structurally extremely stable monolayer, leading to the observed
catalysis of the HER.
Keywords: Reductive SAM desorption; Hydrogen evolution; Catalysis;
Sum frequency generation spectroscopy; Spectroscopic ellipsometry
Self-assembled monolayers (SAMs) are often employed for electrochem-
ical applications such as control of charge transfer [1, 2], production of
(bio)electrochemical sensors [3–5], metal/organic/metal junctions [6–8], and
barrier layers against metal corrosion [9–11]. It is essential to understand the
effect of SAM–modified electrodes on electron transfer reactions, as well as
their interfacial structure under electrochemical conditions for their ultimate
use in electrolytic media.
In most cases, the rate of an interfacial chemical reaction is reduced by
the presence of adsorbates because of their blocking of active sites on the
surface, as has been shown,
e.g., for the oxygen reduction reaction (ORR) on
Au(111) [9, 12, 13]. If the adsorbate blocks the active sites through covalent
bonds, such a rate decrease is also expected for the HER. On the other hand,
redox-active physisorbed monolayers have been shown to act as catalysts
towards the HER [14].
For thiol self-assembled monolayers (SAMs) on Au, HER occurs in an
electrode potential range beyond reductive desorption of the SAMs [15],
leading to a complex interplay between different interfacial reactions. To
understand this complex interplay, it is essential to understand the interfa-
cial properties of SAM-modified electrodes under electrochemical conditions.
In this context, the influence of the electrode potential on formation kinetics
[16, 17], structural properties [18–20], and ionic permeability of SAMs has
been subject to several works [19, 21]. Especially electrochemical desorp-
tion/readsorption studies revealed important details concerning the nature of
the interfacial interactions between a chemisorbed monolayer and a metallic
substrate, besides defining the applicable potential range for SAM-modified
On the cathodic side, this potential range is typically limited by the re-
ductive desorption of thiol-SAMs from the Au substrate, which is commonly
described by the simple reaction [22]
RSAu + e– −→ RS– + Au
Although the cleavage of the Au-S covalent bond has been established, many
aspects of the entire electroreductive desorption mechanism of thiol mono-
layers are unclear and have been subject of a number of studies, in most
cases excluding the effect on and the role of the HER [23–37]. Besides the
currents originating from the Faradaic 1, incidental capacitive currents have
been reported during desorption of a SAM as a consequence of the transition
between a SAM–covered and a bare interface. Cathodic peaks originating
from capacitive currents are usually not larger than 20-30 % of the des-
orption currents, as reported for long-chain aliphatic thiols [23–25, 32–36],
because charges transferred during such capacitive processes are smaller than
the charges transferred during Faradaic reactions.
The state of the molecular units of SAMs during and after electrore-
ductive desorption is decisive for the electrode's activity towards interfacial
reactions, including HER. After electroreductive cleavage of the S-Au bonds,
soluble molecules diffuse into the electrolyte, while long-chain alkanethio-
lates reside in the double layer region as a consequence of their poor water
solubility.[38] Within the double layer, chemically desorbed long-chain alka-
nethiolates form aggregates, as concluded from spectroscopic and microscopic
studies [26, 28, 30, 31]. Depletion of these aggregates has been proposed as
the source of additional capacitive currents, which are usually observed as
a separate peak in voltammograms during desorption of long
n-alkanethiols.
This hypothesis was later disproven by a second-harmonic-generation study
[37]. In addition, vibrational sum-frequency-generation (SFG) spectroscopy
has been applied to investigate molecular ordering and orientation within
self-assembled monolayers at electrified interfaces [39–42], and
ex situ af-
ter treatment at different potentials [38]. The majority of previous studies
of electroreductive desorption focused on commonly used aliphatic SAMs.
In recent years, interest has shifted towards SAMs consisting of aromatic
molecular units, due to their enhanced stability and interesting electric and
electronic properties [1, 2, 43–45]. So far, to the author's knowledge, no de-
tailed desorption study of such technologically promising SAMs composed of
large aromatic molecules with more than two aromatic rings has been car-
ried out. Different in mechanism from multiwave desorption peaks observed
for
n-alkanethiols, long araliphatic thiols show an extraordinary multiwave
response during electroreductive desorption [46]. The corresponding trans-
ferred charges are too large for a capacitive process and even larger than the
Faradaic desorption reaction. The magnitude of the unknown, desorption-
related Faradaic process points to involvement of the HER [46]. A full in-
vestigation of the physical state of the desorbed molecules on the electrode
surface requires spectroscopic or microscopy techniques combined with elec-
This work examines the unusual desorption behavior of the highly ordered
araliphatic thiol monolayers using previously characterized SAMs of (4-(4-
(4 pyridyl)phenyl)phenyl)methanethiol (PyPP1) and 2-(4-(4-(4-pyridyl)phe-
nyl)phenyl)ethanethiol (PyPP2). A series of voltammetric measurements
have been performed to determine the origin of the extraordinarily large
cathodic peaks that appear after reductive desorption. Transient states at the
SAM-Au interface and within the monolayer film and during HER have been
monitored
in situ and
in operando by femtosecond-based broadband SFG
spectroscopy and spectroscopic ellipsometry. Combining the voltammetric
and spectroscopic results, a model is proposed explaining the voltammetric
response of electroreductive desorption of the investigated SAMs.
2.1. Samples
PyPP1 and PyPP2 organothiols were synthesized as described elsewhere
[47]. Au(111) substrates were prepared by evaporating gold onto freshly
cleaved mica sheets at a substrate temperature of 450◦C in a Leybold Uni-
vex 450 system. Prior to use, the Au films were annealed in a H2 flame.
Polycrystalline Au (poly-Au) substrates were mechanically polished. SAMs
were formed by immersion of the Au substrates into 20 µM ethanolic thiol
solutions for 12-15 h. After removal from solution, samples were thoroughly
rinsed with ethanol and purged with nitrogen gas. All chemicals used were
in reagent grade.
2.2. Voltammetry
For all electrochemical measurements a Compactstat potentiostat (Ivium
Technologies, The Netherlands) was employed. Deaerated 0.1 M NaOH so-
lution was used as electrolyte. Cyclic voltammogramms (CVs) on SAM-
modified Au(111) electrodes were measured with a scan rate of 50 mV s-1,
unless stated otherwise. All electrode potentials E herein are referred to an
Ag/AgCl (3 M KCl) reference electrode. For determination of the charges Q
per electrode area transferred during a voltammetric peak, a linear baseline
was set between the vertices of the peaks in the curves of the current densities
j. This baseline was subsequently subtracted from the original j.
For hydrodynamic measurements a rotating disk electrode (RDE) assem-
bly, and a rotating ring-disk electrode (RRDE) assembly (Pine Research
Instruments, USA) were used. In both configurations, disk electrodes con-
sisted of a mirror polished polycrystalline Au disk. In the RRDE tip, around
the poly-Au disk (diameter 5 mm), a Pt ring was placed with 6.5 mm and 7.5
mm inner and outer diameters, respectively. Prior to measurements, both
the ring and the disk electrodes were modified with a PyPP1 monolayer, so
that during the measurement, thiolate adsorption on the ring was prevented.
In this way, any oxidative current detected on the ring could directly be
assigned to H2 oxidation at the applied ring potential (ER = -0.5 V) but
not to oxidative adsorption of thiolates. Collection efficiency of the SAM–
modified Pt ring was determined to be ≈0.05 (compared to 0.43 without
SAM modification).
2.3. Ellipsometry
For spectroscopic ellipsometry measurements, a PyPP1/Au(111) (2 cm x
1.5 cm) sample was placed in a homemade PTFE electrochemical cell with
suitable apertures for incident and reflected light, as well as for a Ag/AgCl
(3 M KCl) microreference electrode and a Pt spiral as counter electrode.
The cell was placed in the beam path of a SE 800 spectroscopic ellipsometer
(Sentech Instruments, Germany) [48, 49]. The ellipsometric angles Ψ and ∆
were measured every ≈5 s for wavelengths λ from 300 to 820 nm during CV
measurements with a scan rate of 5 mV s-1 between 0 V and -1.6 V. The
angles are transformed into the ellipsometric ratio ρ = rp = tan (Ψ) ei∆ with
The ratio of the amplitude reflection coefficients rp and rs for p- and s-
polarized light, respectively, was analyzed using a perturbation approach [51].
The perturbation parameter J1 is related to the transition of the dielectric
function ǫs(z) of the interfacial region perpendicular to the interface in z-
1 − ǫs(z)) (ǫs(z) − ǫ2)
For layer and layer systems with a total thickness small compared to the λ,
ρ is expanded to first order around a step profile in the dielectric function
with ρ0, yielding [51]
ǫ21ǫ2(q1/ǫ1 + q2/ǫ2)2
is the wavevector component parallel to the interface at which a plane wave
impinges under an angle of θ1, while
is the wavevector component perpendicular to the interface in the respective
medium, where index 1 indicates the medium of incidence and 2 indicates
the gold substrate. Literature values have been used for the wavelength-
dependence of the dielectric functions ǫ1 and ǫ2 of the electrolyte [52], and
gold substrate [53, 54], respectively. Eq. 2 can be solved for J1 and used for
a computation of J1 from experimental data.
For measurements of ρ0, E of a bare Au electrode in 0.1 M NaOH was
varied between 0 and -0.5 V. In this range, recorded changes were on the
order of the noise level.
2.4. Sum–Frequency–Generation Spectroscopy (SFG)
The SFG measurements were performed on a home–built SFG spectrom-
eter [55]. Briefly, an etalon–shaped narrowband visible laser beam with
frequency ωVIS fixed at 800 nm and a tunable broadband (100 fs pulse
duration) infrared (IR) laser beam are spatially and temporally superim-
posed at the sample/electrolyte interface. Superposition results in the gen-
eration of a third beam at the sum–frequency of the two incident beams
ωSFG = ωVIS + ωIR. The resulting SFG intensity is
SFG ∝ χ(2) NIRNVIS
χ(2) = χ(2) + χ(2) = χ(2) +
(ωIR − ωk) + iγk
where χ(2) is the second–order susceptibility of the metallic substrate. The
resonant contribution of the second-order susceptibility χ(2), which in this
case originates exclusively from the SAM, is the superposition of a number
of resonances, each with an amplitude Ak, frequency ωk and the phase dif-
ference φk between substrate and resonant response. The damping constant
of the kth-vibration is denoted as γk. NVIS and NIR are the intensities of the
two incident beams. The non–resonant background consisting of contribu-
tions χ(2) of the Au substrate were suppressed by delaying visible and IR
laser pulses by 400 fs [56]. The effects of SAM desorption were finally mon-
itored by centering the IR beam to 1600 cm-1 and recording spectra under
dry air conditions using a purge–box [55]. All spectra were recorded in p
polarizations for all beams (SFG, VIS, and IR). Incident angles for the IR
and VIS beams were adjusted to 55◦ and 60◦, respectively.
In order to perform SFG measurements under E–control in electrochem-
ical media, a thin–layer analysis cell [55] was modified (Fig. 1) by building
electrolyte (0.1 M NaOH) reservoirs at the sides of the CaF2 prism using a
chemically resistive two-component adhesive (X60, HBM Inc., USA). Two
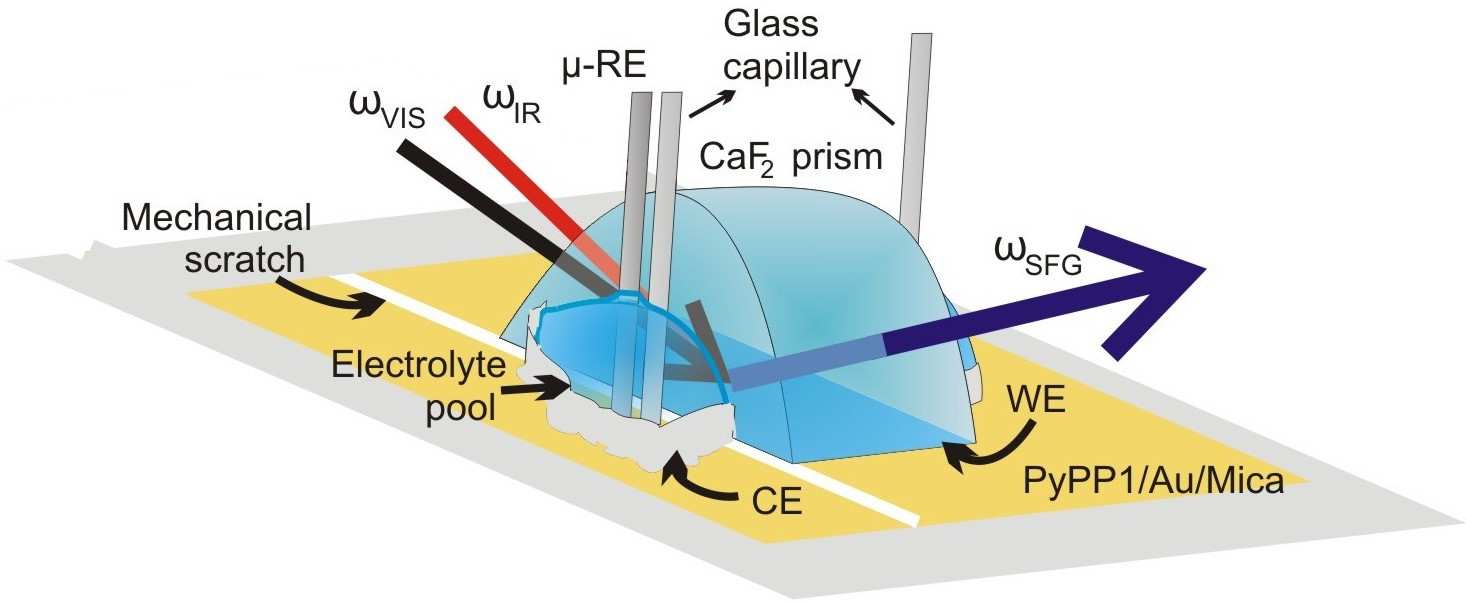
Figure 1: Sketch of the beam path and the arrangement of the electrodes in the modified
thin–layer analysis cell for SFG measurements. The reflected VIS and IR beams are not
glass capillaries containing a part of the electrolyte solution were dipped into
each of the electrolyte pools and slowly released electrolyte into the reser-
voir during the measurements in order to compensate evaporation in dry air
conditions. PyPPx /Au/Mica substrates underlying the prism were extended
over the larger side of the prism into the reservoir. Au films on the substrates
were separated into two parts at the boundary between the prism and reser-
voir by a mechanical scratch so that the part underneath the prism served as
working electrode (WE) and the other part within the reservoir as counter
electrode (CE). An Ag/AgCl (3 M KCl) microreference electrode (RE) was
placed next to the prism in order to minimize the potential drops. In this
configuration, the area exposed to the incident beams was approximately 0.5
cm away from the reference and counter electrodes. The thickness of the
electrolyte layer underneath the CaF2 was estimated to be <1 µm, which
enabled homogenous potential control over the entire sample surface. Right
after the assembly of the electrochemical SFG cell, a series of spectra with an
exposure time of 0.4 s in the background-suppressed mode was started. Af-


j / m
E vs. (Ag/AgCl) / V
-1.8 -1.6 -1.4 -1.2 -1.0 -0.8
-1.8 -1.6 -1.4 -1.2 -1.0 -0.8
E vs. (Ag/AgCl) / V
E vs. (Ag/AgCl) / V
E vs. (Ag/AgCl) / V
Figure 2: First and second CV scan cycles obtained from (a) PyPP1-modified Au(111)
and (b) PyPP2-modified Au(111). In (a) a voltammogram of bare Au(111) is also given
(dashed line). (a◦) and (b◦) show an enlarged view around the cathodic and anodic peaks
of (a) and (b), respectively. (c) PyPP1-modified poly-Au in 0.1 M NaOH. The inset shows
the zoom of the low-current region of the first cycle. Subsequent scans yielded similar
peaks but with smaller intensities as a result of partial readsorption of thiolates.
ter 10 recorded spectra at the open circuit potential (OCP), the potentiostat
was switched on performing a CV starting from the first vertex at 0 V to the
second vertex at -1.6 V with a scan rate of 50 mV s−1. The SFG signal was
then continuously recorded for 10 CV cycles. For obtaining a quantitative
measure of the SFG signal, the recorded background-suppressed spectra were
integrated without any further data treatment.
3. Results and Discussion
3.1. Cyclic Voltammetry (CV)
Fig. 2 presents the CVs obtained for the SAM–modified Au electrodes in
0.1 M NaOH and compares them to the curve for pure Au(111). Adsorption
sites on an Au(111) surface differ for PyPP1 and PyPP2 because of differ-
ent molecular tilt angles arising from the "odd-even" effect involving the
methylene spacers [57]. PyPP1 adsorbs on Au(111) with a (2 3 × 3)R30◦
overlayer structure, whereas PyPP2 yields a (5 3 × 3)rect structure on the
same substrate [57]. The 25% lower surface coverage of PyPP2 on the surface
results in weaker intermolecular interactions compared to PyPP1 [46, 57].
Hence, desorption potentials (C1 peaks in the CVs) of the dilute PyPP2
SAM and the compact PyPP1 SAM differ accordingly. Although C1 peak
potentials vary between PyPP1– and PyPP2–modified Au(111) electrodes,
both systems yield double peaks within the electroreductive desorption re-
gions, as shown in Fig. 2a and b, respectively. For the PyPP1/Au(111)
electrode, the C1 peak at -1.30 V is followed by a much larger second peak
C2 at -1.47 V. By integration of these peaks, Q ∼-0.06 mC cm-2 for C1
and -1.32 mC cm-2 for C2. For desorption of a PyPP1 SAM with the given
overlayer structure on Au(111), a total Faradaic charge of ∼-0.06 mC cm-2
is expected according to Faraday's Law for a single electron transfer process,
Γ = Q/F , where Γ is the surface coverage, and F is the Faraday constant.
The experimental results show the major portion of the theoretically esti-
mated charge for desorption of a complete monolayer to be reached during
the C1 peak. Remarkably, the integrated current of the C2 peak corresponds
to a 22–times larger reductive charge, which results obviously from a Faradaic
process other than thiol desorption. The peak current, hence also the reac-
tion rate, in this second cathodic peak is ∼10 times higher than the current
at the same potential on bare Au(111) during the first cycle, and is still ∼3
times as high as bare Au(111) during the second cycle. In the voltammogram
of the PyPP2/Au(111) electrode, the C1 peak at -1.11 V yields Q ∼-0.06 mC
cm-2, similar to PyPP1/Au(111). Subsequently, a much less pronounced C2
peak appears at -1.42 V with Q ∼-0.01 mC cm-2. A direct relationship be-
tween monolayer structure and the rate of the concomitant Faradaic reaction
is reflected in the CVs of compact PyPP1 and non–compact PyPP2 SAMs on
Au(111). According to a model, compact monolayers such as PyPP1 desorb
via a homogeneous reduction process all over the electrode surface, whereas
etching centers are created in more permeable monolayers and desorption
propagates successively at the edges of these etching centers [58, 59]. The
lower ionic permeability expected for PyPP1 is supposed to cause slower dif-
fusion of counter ions (here Na+) from the electrolyte to the sulfur heads
through the thiols, consequently shifting the desorption potential to more
negative values [46].
The observed extraordinary voltammetric response upon the electrore-
ductive desorption of PyPP1 SAMs becomes even more striking when the
monolayer is adsorbed on a poly-Au surface instead of Au(111). For the
PyPP1/poly-Au electrode, again a double peak is observed in the voltam-
mogram (Fig. 2c). However, the C'1 peak is barely visible next to the massive
C'2 peak. (Here, the peaks are designated as C'1 and C'2 to stress the slight
differences in origin of the peaks.) A Q ∼-0.01 mC cm-2 for the first peak
at -1.15 V suggests that in this peak, only a small portion of the monolayer
desorbs, which most likely consists of weakly bound thiols, e.g. at domain
boundaries. The residual major portion of the monolayer desorbs at more
negative potentials in the dominant second peak at -1.61 V. Integration yields
Q ∼-2.30 mC cm-2 for C'2. Obviously, on a poly-Au substrate, the PyPP1
SAM desorbs at more cathodic potentials compared to an Au(111) substrate.
At the same time, larger reductive currents are observed. The negative po-
tential shift for desorption of the monolayer on a poly-Au substrate compared
to Au(111) can be explained by the increased electrochemical stability of the
monolayer on the polycrystalline surface. It is well-known that the energy
required to desorb SAMs from a gold surface is minimum for Au(111) [60–
63]. Assuming that the poly-Au substrate has a considerable proportion of
low index surfaces other than (111), desorption at more negative potentials
is quite reasonable. However, it is astonishing that by using a poly-Au sub-
strate, the additional Faradic reaction overlaps with the desorption reaction,
yielding a 75% larger Q compared to the PyPP1/Au(111) electrode.
Further CV measurements at different scan rates (not shown, see also
Section 3.3) indicate that the C2/C'2 peak is related to a diffusion–controlled
reaction at the corresponding peak potential. On the other hand, the area
under the C1/C'1 peak (i.e. the charges) is almost independent of the scan
rate, as expected for a peak purely related to desorption.
The CV results show that the main body of the monolayer desorbs from
a poly-Au substrate within the range of the C'2 peak. However, for the
PyPP1/Au(111) system, both peaks are large enough to contain desorption
currents of a monolayer so that the origins of the corresponding peak cur-
rents cannot be distinguished. For determining the beginning and the end
of the monolayer desorption process on this system, changes at the elec-
trolyte/PyPP1/Au interface during a CV scan were tracked using ellipsom-
(b) −1.5
E / V (Ag AgCl)
0 −1.5 −1 −0.5 0
E vs. (Ag/AgCl) / V
Figure 3: Real part of J1 averaged from 340-360 nm (◦, scale on the left, see text forrationale of wavelength range) during (a) the first and (b) the second CV scan (—, scale
on the right) of PyPP1/Au(111) in 0.1 M NaOH. (a) includes Re(J1) (--) on Au(111)
without SAM. Arrows indicate the respective potential scan direction. Scan rate: 5 mV
3.2. Ellipsometry
Fig. 3a shows the real part of J1 as function of E during the first CV scan
of a freshly prepared PyPP1/Au(111) sample. Different from the previously
presented CV measurement (Fig. 2a), in this experiment the scan rate was set
to 5 mV s−1 for achieving a good synchrony between the applied potentials
and ellipsometric data acquisition. A significant decrease is observed in the
current of the second peak compared to the preceding first peak at this low
Taking a look at the corresponding ellipsometry data, a stable and con-
stant Re(J1) is observed until the onset of the C1 peak. The magnitude
observed of Re(J1) ∼ −1 nm in the given wavelength range is expected for
an organic layer of a thickness of ∼1.5 nm, consistent with an intact SAM.
The wavelength–dependence of the data is mainly given by the wavelength–
dependence of ǫ2, which means that the curves appear similar in shape but
different in magnitude at all wavelengths. The wavelength range displayed
here was chosen as it is the region where ǫ2 is closest to 0, which simplifies the
analysis according to Eq. 1. At the onset of the C1 peak in the CV, Re(J1)
increases and reaches zero approximately in the middle of the desorption
peak. Re(J1) = 0 corresponds to a complete absence of a layer, or the can-
cellation in Eq. 1 of the effect of regions with positive or negative dielectric
constant contrast. Re(J1) continues to rise above zero until the onset of the
C2 peak, and remains constant with further increasing cathodic potentials
until the potential vertex at -1.6 V. The positive value of Re(J1) implies that
the dominating layer in the interfacial refractive index profile now has a real
part of the dielectric constant at the respective frequency between the value
of the electrolyte (∼1.8) and Au (∼-1.1), which is untypical for organic mate-
rials. During the reverse scan, re-adsorption is observed as a slight decrease
in Re(J1), which remains at values >0. Readsorption is barely visible in the
CV current, because the scan rate is not high enough to display respective
charges. The results obtained during the subsequent second scan are shown
in Fig. 3b. In the direction of increasing cathodic potential, Re(J1) resembles
closely the backward scan of Fig. 3a. Desorption is barely visible in Re(J1),
but it is in the CV. In the backward scan, values of Re(J1) approach more
and more the value of 0. The increased fluctuations in Re(J1) in the later
stages of the experiment are attributed to the presence of H2 bubbles, which
remain on the surface after E is entering the regime of HER for the first time,
and the consequent increase in scattered light intensity on the expense of the
detected reflected intensity. It must be pointed out that the changes in the
curves between the different scans are not due to drift of the instrument, but
are caused by genuine changes in the interfacial structure. Corresponding
experiments with bare Au show highly repeatable scans.
The presented ellipsometry results reveal information about the state
of the PyPP1/Au interface during electrochemical polarization. The main
change in Re(J1) is observed during the first forward CV scan between the
C1 and C2 peaks. In this potential regime, desorption of the SAM occurs,
however, its constituents remain present near the interface. During subse-
quent readsorption, Re(J1) is still far away from its initial value and even
shows opposite sign to the initial value. One might expect that the SAM
loses all order during the desorption process, but SFG measurements, which
will be discussed later, show that order is maintained in the course of des-
orption, and is also maintained over at least 10 CV cycles. The most likely
explanation for the large effect in the first scan compared to all other is that
desorption leads to the presence of a layer or patches of low refractive index
between SAM and Au surface or between SAM and electrolyte. The value of
Re(J1) ∼ 2 nm after the desorption can be explained by the presence of an
effectively ∼2.6 nm thick layer of a material with ǫs = 1, if a total loss of the
SAM is assumed. As the SAM is still present, the effective layer thickness
must be even higher. The presence of macroscopic H2 bubbles, which are
visible below -1.4 V, cannot account for the observed effect. Macroscopic
bubbles barely affect Re(J1), as has been confirmed in control experiments
in the absence of a SAM. Results from one control experiment are shown in
Fig. 3a. The aforementioned layer of low refractive index is likely in part
to be consisting of adsorbed H2, which starts to form below the desorption
potential. As not all gas is removed from the system when scanning in an-
odic direction, it may remain adsorbed, explaining the large and irreversible
shift to positive values of Re(J1). Further, desorption may also lead to sub-
stantial changes in the electronic structure of the Au surface, resulting in
respective irreversible changes of the dielectric function of Au near the inter-
face. A third possibility is a substantial change in solvent structure around
the desorbed SAM. This last explanation is, however, unlikely, because even
relatively large changes result only in rather weak effects on the dielectric
constant at optical frequencies.
It is also worth to underline an important result from the comparison of
the ellipsometric and CV results. The intensities of the two voltammetric
peaks and the degree of shift in Re(J1) do not match. This observation
indicates different mechanisms as the sources of the two subsequent cathodic
peaks on the voltammograms of PyPP1/Au(111) samples. Consequently, the
first reductive peak can be assigned mainly to the desorption of the SAM.
In order to elucidate the origin of the large excess currents appearing as the
second peak on PyPP1/Au(111) and overlap with the desorption currents
inside the same peak on PyPP1/poly-Au systems, further investigations were
done by hydrodynamic voltammetric measurements.
3.3. Hydrodynamic voltammetry
So far, the observed excess cathodic charges were postulated to result
mainly from a parallel Faradaic reaction concomitant to desorption of a
PyPP1 monolayer. HER is a likely candidate as the possible source of the
generated currents, because these excess currents are observed at potentials
where HER is thermodynamically possible [46]. In order to confirm the role
of H2, RRDE measurements were performed using a PyPP1/poly-Au disk
electrode surrounded by a Pt ring, which was also modified with PyPP1
monolayer without extermination of the H2 sensitivity. Fig. 4 shows the
voltammograms for the ring and disk electrodes at 900 RPM angular rotation
speed in 0.1 M NaOH. Both the ring and the disk voltammograms look quite
symmetrical. Similar to the CV shown in Fig. 2c, the PyPP1/poly-Au disk
electrode shows a desorption-related large cathodic peak covering a potential
range between -1.4 V and -1.7 V. On the ring electrode, a corresponding
oxidative peak is observed. After correction of the ring voltammogram re-
garding its collection efficiency, the absolute value of the charge underneath
the anodic peak on the ring corresponds to ∼90% of the of the cathodic
peak charge on the disk voltammogram. The relation between the ring and
/ m
j R
/ m
j D
E vs. (Ag/AgCl) / V
E vs. (Ag/AgCl) / V
Figure 4: (a) RRDE data. Voltammograms obtained from PyPP1/poly-Au disk (jD) and
PyPP1/Pt ring (jR) during the first scan cycle in 0.1 M NaOH with 900 RPM angular
rotation speed and 50 mV s−1 scan rate. Ring potential was fixed at -0.5 V. (b) RDE
data. Voltammograms of PyPP1/poly-Au electrode in 0.1 M NaOH at various angular
rotation speeds ω. The inset shows the reductive charge density vs.
disk currents is only approximate, because the determination of the collec-
tion efficiency of a thiol modified ring is prone to errors. Nonetheless, since
readsorption of released thiolates on the thiol modified ring can be ruled out,
this anodic peak can be assigned mainly to oxidation of H2 that is formed on
the disk electrode concomitant to desorption of the monolayer. The observed
C2 and C'2 peaks in the CVs can therefore be related to the evolution of H2.
Because in this peak, the HER is significantly faster than on bare Au, the
term "rapid HER" will be used here to described this special kind of HER.
In order to investigate the relationship between HER kinetics and SAM
desorption, further hydrodynamic voltammetry experiments were carried
out using identical PyPP1/poly-Au electrodes at varying electrode angu-
lar rotation speeds. A series of the hydrodynamic voltammograms for the
PyPP1/poly-Au electrodes is presented in Fig. 4b. At each rotation speed the
cathodic peak corresponding to C'2 is visible. However, the peak potential
of the large peak gradually shifts to more anodic potentials with increas-
ing rotation rates. Under hydrodynamic conditions, after cleavage of the
S-Au bonds, thiolates are expected to diffuse faster away from the double
layer region at high rotation speeds, as shown in previous experiments [64].
The transport of H2 away from the electrode is also likely to contribute to
transport of thiolates away from the electrode surface. At slower rotation,
thiolates suspended in the double layer should slow down desorption of the
remaining thiols [64]. Therefore, a SAM desorbs at slightly more anodic po-
tentials when the electrode rotation speed is increased. For the investigated
PyPP1/poly-Au electrodes, the total potential shift amounts to 60 mV (from
-1.61 V to -1.55 V) between 0 and 4000 RPM. Due to the association between
SAM desorption and rapid HER, the reductive peaks shift without chang-
ing their shape. Interestingly, peak charges decrease with increasing angular
rotation speed (inset in Fig. 4b). These results indicate that retention time
of the desorbed PyPP1 molecules has an influence on the HER kinetics. At
this point, it becomes critical to know in what structural state the thiolates
reside above the Au surface after desorption. The following experiment using
vibrational SFG spectroscopy addresses this question.
3.4. SFG vibrational spectroscopy
For monitoring order of thiol/thiolate molecules during voltammetric
scans, SFG spectroscopy was applied both in situ and in real time. Measure-
ments were performed using Au(111) substrates, because flat surfaces are re-
quired to obtain adequate spectroscopic data. In addition to PyPP1/Au(111),
a PyPP2/Au(111) sample was investigated for comparison, which has a dif-
ferent structural quality and voltammetric response with less pronounced
additional Faradaic contributions, as shown in Fig. 2.
Fig. 5a shows the first one and a half voltammetric cycles of a typical
SFG experiment. The spectrum shows an SFG band attributed to the ring
vibration of the pyridine moiety of PyPP1, centered around 1600 cm−1 [57].
This mode was chosen because a measurement of the full SFG spectrum has
shown this band to be extremely intense. This extremely high intensity is
required for real-time measurements during the CVs. The evolution of the
signal intensity over time is alternating presumably due to nonlinear χ(3)
effects related to the coupling of visible, IR and static electric field at the
interface, which is discussed in detail elsewhere [65]. No further significant
change is observed in the intensity curve during the voltammetric scan, not
even in the peak potential regions below -1.25 V. This stable alternating sig-
nal indicates that the two–dimensional crystalline order of the monolayer is
persevered at all applied potentials. The SFG signal obtained from ordered
PyPP1 structures is not necessarily acquired only from the thiols directly
present on the Au(111) surface but also from the surface plane of the electri-
cal double layer. The first two CV scans recorded in parallel of SFG spectral
aquisition are shown in 5 b. The curves are slightly different compared to
those in Fig. 2, because of the different cell geometry. Especially the read-
sorption peak is more pronounced. Overall, CV data shows that after each
cycle, the amount of the readsorbed molecules becomes smaller. Hence the
observed stable SFG signals comprise contributions of both adsorbed and
suspended molecules as long as they remain ordered. In Fig. 5c the nor-
malized integrated SFG signals are plotted as function of the number of CV
cycles the electrode was subjected to. Because the intensity of the signal is
related to the order, number density, and orientation of molecules, a decrease
in the maximum intensity value indicates loss in the structural molecular ar-
rangement within the surface plane. Astonishingly, the signal obtained from
PyPP1 does not show significant variations after 10 voltammetric scan cy-
cles between 0 V and -1.6 V. On the other hand, the intensity for PyPP2
decreases continuously after each cycle. After 10 cycles, the PyPP2 signal
has almost vanished completely due to loss of order and/or decreased num-
ber density within the SAM. After leaving the PyPP1 monolayer at open
circuit for ∼30 min after desorption experiments, the signal of PyPP1 has
also completely disappeared, indicating a diffusion of the molecules from the
surface into the bulk.
It is worth noting that readsorption peaks in the CVs of Section 3.1,
as well as those measured in the CVs during SFG experiments, are small,
considerably smaller than the desorption peaks. In a previous work, read-
sorption was quantified of PyPP1 and PyPP2 between -0.2 and -1.8 V [46]:
25 % of PyPP1 and ∼50 % of PyPP2 readsorb after each cycle. However,
the molecules remain physisorbed to the electrode surface, even though the
Au-S bond is broken. SFG vibrational spectra do not distinguish between
ordered layers covalently bound to Au and ordered layers physisorbed to Au.
Therefore, the presence in the SFG spectra of the characteristic vibrational
mode from the molecules shows layer order, and hence also PyPPx presence,
in the interfacial region.
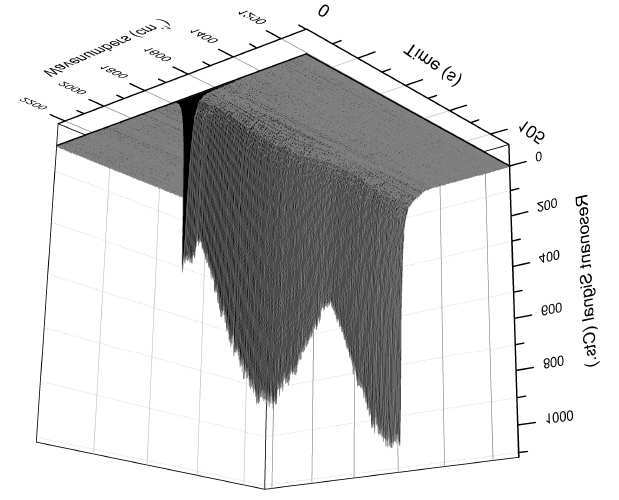
I / m -0.2
E vs. (Ag/AgCl) / V
er / c 1400
Figure 5: (a) Evolution of a pyridine ring vibration of PyPP1 on Au(111) during potential
sweeps from 0 V to -1.6 V, back to 0 V, to finally -1.6 V, corresponding to one and a
half CV cycles, as measured with SFG vibrational spectroscopy. (b) First two CV scans
recorded in the SFG cell during aquisition of spectra shown in (a). (c) Integrated SFG
intensity of the observed band from PyPP1/Au(111) and PyPP2/Au(111) during ten CV
cycles. The integrated SFG signals are normalized to the maximum signal obtained from
an ordered SAM for each thiol.
3.5. Discussion of rapid HER origin
The combination of the voltammetric and spectroscopic results obtained
from PyPP1 and PyPP2 modified Au samples reveals two important features
that make the desorption process especially of PyPP1 extraordinary com-
pared to the desorption of any aliphatic or short araliphatic thiol reported
to the author's knowledge. The first extraordinary feature is the preserva-
tion of two–dimensional order of the reductively desorbed thiolates within
the double layer region, that exists over a long period of time and a large
potantial range after desorption. The second feature is the association of
rapid HER with the desorption of the SAMs. These two features are closely
related, considering the observed differences in desorption between PyPP1
and PyPP2 SAMs. The correlation between these features helps to interpret
the complicated voltammetric desorption behavior on a solid ground.
Previous studies with aliphatic thiols showed that the structure of the
desorbed thiols undergoes a transition from upright molecular oriented films
to aggregates [26, 27, 29, 30, 66], or a striped phase with flat lying dithiol
molecules [67], in the electrochemical double layer region. Based on subtrac-
tively normalized interfacial infrared spectra [27, 66], and in situ scanning
tunneling microscopy studies [26], aggregates of long n-alkanethiolates have
been proposed to be in form of micelles. The micelle formation process
involves a competition between repulsive forces among negatively charged
sulfur head groups, attractive van der Waals forces among the chain units,
and hydrophobic forces. Hydrophobic termination groups accelerate micelle
formation. Taking all attractive and repulsive contributions into account, the
shape of the aggregates can be estimated from the semiempirical "packing
parameter" v/(a0lc) involving the optimal surface area a0 of the molecu-
lar head group, chain length lc, and chain volume v [68]. For hydrocar-
bon amphiphiles, micelle formation becomes favorable if v/(a0lc) < 1/3 [68].
Due to the small v/(a0lc) of the long aliphatic thiols with conformational
disorder in the desorbed state, micelle formation is conceivable for the n-
alkanethiolates. On the other hand, as the araliphatic chain is rather rigid,
araliphatic molecules exhibit a shape close to a cylinder, where v/(a0lc) ∼ 1.
This value of v/(a0lc) implies favorable formation of planar aggregate struc-
tures. Consequently, loss of crystalline order after reduction is expected to
occur slower for long araliphatic thiols. According to the SFG spectroscopy
measurements, for the thiolates of PyPP1 the crystalline structures inherited
from the chemisorbed monolayer are preserved in both voltammetric peak re-
gions (Fig. 5). According to the structural differences between PyPP1 and
PyPP2 monolayers, an even more stable ordered thiolate structure is ex-
pected for the compact PyPP1 SAM, which is confirmed by the presented
SFG results. These results are clear indication of the astonishingly long life-
time of the ordered thiolate structures for the investigated SAMs, especially
Apart from the stable structural order of the desorbed thiolates, a sec-
ond striking result is the detection of HER concomitantly to desorption of
the PyPP1 monolayer. On poly-Au, a rapid HER was found to occur si-
multaneously with the desorption of the main fraction of the SAM. On the
other hand, based on the ellipsometry results, these two reactions are not
simultaneous on Au(111) surfaces, were HER is found to occur only after
desorption of the SAM. Both processes and are observed as separate peaks
in voltammograms (Fig. 3). The first reductive peak originates mainly from
SAM desorption, as confirmed by shift in Re(J1) at the corresponding po-
tentials. Because no pronounced shift in Re(J1) is detected in the potential
region of the subsequent large peak, it can be deduced that this peak consists
mainly of HER currents. When the rapid HER starts, the surface is already
saturated with H2, so the main fraction of the additionally produced H2 is
leaving the system as gas, therefore it is not present in the interfacial region.
This finding can be generalized for the other members of the araliphatic thiol
series, including PyPP2, which also yields excess cathodic currents in parallel
to the thiol reduction process as observed on Au(111) surfaces.
Considering the results of the RDE measurements, a relationship between
the rate of the HER (hence, catalytic activity of a surface towards HER) and
the thiolate's lifetime in the surface plane of the electrode is observed. The
presented voltammograms obtained from PyPP1/poly-Au electrodes (Fig.
4b) show that peak currents become smaller at faster electrolyte convection
rates. Smaller peak currents in case of shorter lifetime of thiolates in the
double layer region indicate a catalytic activity on the Au electrode through
the presence of desorbed thiolates. A similar behavior was observed with
PyPP1/Au(111) electrodes; the second H2-related peak became smaller com-
pared to the first desorption-related peak when the CVs were recorded with
a lower scan rate (comparing Fig. 2a and Fig. 3a). Altogether these observa-
tions indicate a diffusion–related phenomenon. The catalytic effect observed
for HER requires the presence PyPP1 monolayers on the electrode surface
without covalent bond to the electrode.
The above–stated arguments lead to models for the overall desorption
mechanism of the investigated thiols. First, consider the desorption of PyPP1.
As shown in Reaction 1, thiol desorption requires exchange of electrons be-
tween Au substrate and thiols. This reaction, however, involves also the
substitution of thiol molecules with water on the Au surface [35],
(ads) + ne– + xH2O −→ nRS(aq) + (x−y)H2O(ads).
Since Reaction 2 (where y stands for the number of water molecules required
for the solvation of the thiolate) occurs at sufficiently negative potentials for
HER, the adsorbed water layer is immediately consumed for formation of
2O(ads) + e– −→
Reactions 2 and 3 are reactions that occur for any type of thiol on an Au
surface. It must be pointed out that the participation of HER in the desorp-
tion process leads to an current contribution also, which may account for the
fact that the integrated desorption currents are slightly higher than required
for monolayer desorption. In this specific case, especially for desorption of
PyPP1, the question arises: What is the reason of the temporary acceleration
of Reaction 3? In the following, several possible models will be discussed.
The overall model is schematically summarized in Fig. 6.
One possible reason for high HER rates associated with desorption is the
retarded surface reconstruction of the Au substrate. It is currently widely
accepted that thiolate bonding on Au results in a lower density of Au surface
atoms compared to the SAM–free surface. SAM formation involves displace-
ment of Au atoms on the surface; lifting the Herringbone reconstruction and
formation of vacancy islands (etch pits). Recent studies demonstrated that
Figure 6: Schematic view of the proposed model for the rapid HER. (a) shows the intact
SAM, which is reductively desorbed (b). Subsequent transport of water into the region
between desorbed monolayer and Au leads to H2 evolution (c).
together with vacancy islands, additional Au adatoms are formed, which serve
as energetically favoured bonding sites for thiolates [69–71]. Compared to a
reconstructed surface, such a modified Au surface underneath the SAM may
offer more catalytically active sites for reactions such as HER, if it becomes
accessible to the electrolyte. However, it has been reported for alkyl-thiols
that desorption of the SAM immediately initiates surface reconstruction, in-
cluding formation of the herringbone structure on Au(111), roughening of
step edges, formation of gold islands and disappearance of vacancy islands
[30, 72–74]. However, because the adsorption of the aliphatic and araliphatic
thiols result in the same changes on an Au surface [43, 57], a similar recon-
struction mechanism is expected for both thiol types after reductive desorp-
tion. For aliphatic thiols, no increase in the HER rate is, however, observed.
As a result, a substantial difference on the Au surface after reductive desorp-
tion of aliphatic and araliphatic SAMs is unlikely but cannot be completely
ruled out here.
Catalysis of HER through physical interactions between the Au surface
and any units of the desorbed thiolates, e.g., the pyridine moiety, can be
safely ruled out as a possible explanation, due to the orientation of thiolates
in the related potential region. In this orientation, only the sulfur heads are
facing to the Au surface, separated from the Au surface by an electrolyte gap
of ∼1 nm [75, 76]. Assuming a similar separation distance for PyPP1 thio-
lates, a strong influence of the ordered, suspended thiolates on the structure
of the electrolyte between Au and desorbed SAM is expected. In any assem-
bly form, the suspended negatively charged thiolates are prone to interactions
with counter ions in the solution. Protonation of electroreductively desorbed
n-alkanethiolates has been suggested despite the high solution pH [77, 78].
However, at pH 13, protonation of the full monolayer is not expected, as
the pKa values for HS-R units are usually lower,[78] e.g. for C6H5-CH2-SH,
The most likely mechanism for the observed acceleration of the HER after
reductive desorption of the thiol SAM involves orientation of water molecules
in the gap between Au and suspended monolayer. Diffusion of positively
charged counter ions, in this case Na+ , from the electrolyte is essential
for desorption of SAMs [77]. If the desorbed thiolates form aggregates, a
homogenous charge distribution on the Au surface is quickly established.
On the other hand, if the organic layer does not lose its two-dimensional
order after desorption, as observed here, Na+
will be present in a hydrated
form between the negatively charged thiolate and the negatively polarized
Au surface (Fig. 6b). This additional layer of hydrated positive ions in the
gap between the organic layer and the Au surface is suggested here to lead
to an optimum pre-orientation of water for a participation in the HER, and
consequently higher HER rates (Fig. 6c). The ideal orientation of water
needed for participation in the HER is not known. Two possible candidates
are marked in Fig. 6c, though to achieve direct experimental evidence is
The suggested model for the catalysis of HER — though speculative at
this point — can be employed to explain the double peaks observed on the
voltammograms upon desorption of the SAMs. For the catalytic activity
through water reorientation, transport of Na+
from bulk solution into the
gap between the thiolate monolayer and the Au surface is required. PyPP1
thiolates with orientation as observed are not prone to electrolyte penetra-
tion through the suspended film. Water and ion transport from the bulk
electrolyte into the volume between Au and suspended monolayer occurs
through inherent structural defects in the organic monolayer, such as do-
main boundaries. On an Au(111) surface, after consumption of the initially
adsorbed water layer, further water transport into the inner plane requires
additional time due to the existence of large electrolyte–blocking SAM do-
mains and a low amount of defects in the thiolate layer. Therefore, currents
from the catalyzed HER are seen in a peak that is separated from the thiol
reduction peak (Fig. 2a), and in general currents show a diffusion–related
behavior in the electrochemical experiments. On poly-Au, due to the higher
amount of structural defects in the SAM, electrochemical desorption and
subsequent transport of electrolyte into the gap occur much faster, so that
currents from both Faradaic reactions overlap (Fig. 2c). With increasing
amount of water molecules in the gap between the suspended thiolate layer
and the Au surface, contribution of HER to the peak currents increases. In
the proposed mechanism, depletion of thiolate molecules causes loss of the
observed catalytic activity, because the optimum distribution of Na+
pends on the existence of the oriented thiolates in the double layer. Because
the suspended thiolate layer also hinders the transport of the produced H2
gas from the Au surface, the observed rate increase does not last long. In
addition to formation of macroscopic bubbles, which are observed at strongly
cathodic potentials only, the formed H2 can be adsorbed either between Au
and suspended SAM, or at the SAM/electrolyte interface.
An accelerated ("rapid") HER has been detected concurrent to desorp-
tion of structurally stable PyPP1 SAMs. The rate of the HER is strongly
influenced by the structure of desorbed thiolates residing near the electrode
surface after desorption. In situ ellipsometry results show a decrease of the
interfacial refractive index upon SAM desorption. Combined electrochemical
and SFG spectroscopy investigations show that after electroreductive desorp-
tion, the structural order of the araliphatic thiol films is inherited from the
chemisorbed state. For the compact PyPP1-SAM the two-dimensional or-
der is astonishingly stable. Comparison with the less compact PyPP2-SAM,
where two–dimensional order is not persevered upon reductive desorption, as
well as with literature data on aliphatic SAMs, shows that the structural sta-
bility of the desorbed SAM is a prerequisite for the occurrence of the increase
in HER rate.
The results imply that the presence of a highly ordered adsorbed layer of
non–redox active species can increase, rather than decrease, the rate of an
interfacial electron transfer reaction, in this case of the HER, here likely due
to the ordering effect of the organic monolayer on the solvent and reagent wa-
ter. The ordering effect occurs via the transient species of thiolates residing
in the double layer region immediately after cleavage of the Au-S bond. The
stability of the SAM after desorption is crucial for the observed effect. The
exact nature of the interfacial water requires detailed investigations from a
combination of vibrational spectroscopies and molecular simulations.
M.I.M. thanks the IMPRS SurMat for a scholarship. A. Terfort gener-
ously provided the substances for this study. M. Stratmann is acknowledged
for his continuous support. C.B. and P.K. acknowledge support from the
Helmholtz Program BioInterfaces and P.K. from NIH Grant EB-002027 to
the National ESCA and Surface Analysis Center for Biomedical Problems.
Y. C. thanks for support from the European Union and the state of North
Rhine-Westphalia in the frame of the HighTech.NRW program.
[1] S. B. Sachs, S. P. Dudek, R. P. Hsung, L. R. Sita, J. F. Smalley, M. D.
Newton, S. W. Feldberg, C. E. D. Chidsey, Rates of interfacial electron
transfer through π-conjugated spacers, J. Am. Chem. Soc. 119 (1997)
[2] D. M. Adams, L. Brus, C. E. D. Chidsey, S. Creager, C. Creutz, C. R.
Kagan, P. V. Kamat, M. Lieberman, S. Lindsay, R. A. Marcus, R. M.
Metzger, M. E. Michel-Beyerle, J. R. Miller, M. D. Newton, D. R. Roli-
son, O. Sankey, K. S. Schanze, J. Yardley, X. Zhu, Charge transfer on
the nanoscale: current status, J. Phys. Chem. B 107 (2003) 6668.
[3] N. K. Chaki, K. Vijayamohanan, Self-assembled monolayers as a tunable
platform for biosensor applications, Biosens. Bioelectron. 17 (2002) 1.
[4] J. Gooding, F. Mearns, W. Yang, J. Liu, Self-assembled monolayers into
the 21st century: Recent advances and applications, Electroanalysis 15
[5] M. Frasconi, F. Mazzei, T. Ferri, Protein immobilization at goldthiol
surfaces and potential for biosensing, Anal. Bioanal. Chem. 398 (2010)
[6] T. Baunach, V. Ivanova, D. Kolb, H.-G. Boyen, P. Ziemann, M. B¨
P. Oelhafen, A new approach to the electrochemical metallization of
organic monolayers: Palladium deposition onto a 4,4-dithiodipyridine
self-assembled monolayer, Adv. Mater. 16 (2004) 2024.
[7] C. Silien, D. Lahaye, M. Caffio, R. Schaub, N. R. Champness,
M. Buck, Electrodeposition of palladium onto a pyridine-terminated
self-assembled monolayer, Langmuir 27 (2011) 2567.
[8] M. I. Muglali, J. Liu, A. Bashir, D. Borissov, M. Xu, Y. Wang, C. Woll,
M. Rohwerder, On the complexation kinetics for metallization of or-
ganic layers: palladium onto a pyridine-terminated araliphatic thiol film,
Phys. Chem. Chem. Phys. 14 (2012) 4703.
[9] M. Rohwerder, M. Stratmann, Surface modification by ordered mono-
layers: New ways of protecting materials against corrosion, MRS Bull.
24 (1999) 43.
[10] J. Mathiyarasu, S. Pathak, V. Yegnaraman, Review on corrosion pre-
vention of copper using ultrathin organic monolayers, Corros. Rev. 24
[11] F. Caprioli, F. Decker, A. G. Marrani, M. Beccari, V. D. Castro, Copper
protection by self-assembled monolayers of aromatic thiols in alkaline
solutions, Phys. Chem. Chem. Phys. 12 (2010) 9230.
[12] M. I. Muglali, A. Bashir, M. Rohwerder, A study on oxygen reduc-
tion inhibition at pyridine-terminated self assembled monolayer modified
Au(111) electrodes, Phys. Status Solidi A 207 (2010) 793.
[13] A. Nowicka, U. Hasse, G. Sievers, M. Donten, Z. Stojek, S. Fletcher,
F. Scholz, Selective knockout of gold active sites, Angew. Chem., Int.
Ed. 49 (2010) 3006.
[14] T. Uchida, H. Mogami, A. Yamakata, Y. Sasaki, M. Osawa, Hydro-
gen evolution reaction catalyzed by proton-coupled redox cycle of 4,4-
bipyridine monolayer adsorbed on silver electrodes, J. Am. Chem. Soc.
130 (2008) 10862.
[15] R. Alkire, D. Kolb, J. Lipkowski, P. Ross, Chemically Modified Elec-
trodes, Wiley-VCH, Weinheim, 2009.
[16] M. Rohwerder, K. de Weldige, M. Stratmann, Potential dependence of
the kinetics of thiol self-organization on Au(111), J. Solid State Elec-
trochem. 2 (1998) 88.
[17] F. Ma, R. B. Lennox, Potential-assisted deposition of alkanethiols on Au:
Controlled preparation of single- and mixed-component SAMs, Lang-
muir 16 (2000) 6188.
[18] M. J. Esplandi´
u, H. Hagenstr¨om, D. M. Kolb,
Functionalized self-
assembled alkanethiol monolayers on Au(111) electrodes: 1. Surface
structure and electrochemistry, Langmuir 17 (2001) 828.
[19] N. Darwish, P. K. Eggers, S. Ciampi, Y. Zhang, Y. Tong, S. Ye, M. N.
Paddon-Row, J. J. Gooding, Reversible potential-induced structural
changes of alkanethiol monolayers on gold surfaces, Electrochem. Com-
mun. 13 (2011) 387.
[20] J. Zhang, A. C. Welinder, Q. Chi, J. Ulstrup, Electrochemically con-
trolled self-assembled monolayers characterized with molecular and sub-
molecular resolution, Phys. Chem. Chem. Phys. 13 (2011) 5526.
[21] W. Wang, S. Zhang, P. Chinwangso, R. C. Advincula, T. R. Lee, Electric
potential stability and ionic permeability of sams on gold derived from
bidentate and tridentate chelating alkanethiols, J. Phys. Chem. C 113
(2009) 3717.
[22] C. A. Widrig, C. Chung, M. D. Porter, The electrochemical desorption
of n-alkanethiol monolayers from polycrystalline Au and Ag electrodes,
J. Electroanal. Chem. Interfacial Electrochem. 310 (1991) 335.
[23] T. W. Schneider, D. A. Buttry, Electrochemical quartz crystal microbal-
ance studies of adsorption and desorption of self-assembled monolayers
of alkyl thiols on gold, J. Am. Chem. Soc. 115 (1993) 12391.
[24] P. Krysinski, R. V. Chamberlain, M. Majda, Partial electron transfer
in octadecanethiol binding to gold, Langmuir 10 (1994) 4286.
[25] D.-F. Yang, C. P. Wilde, M. Morin, Electrochemical desorption and
adsorption of nonyl mercaptan at gold single crystal electrode surfaces,
Langmuir 12 (1996) 6570.
[26] D. Hobara, K. Miyake, S.-I. Imabayashi, K. Niki, T. Kakiuchi, In-
situ scanning tunneling microscopy imaging of the reductive desorption
process of alkanethiols on Au(111), Langmuir 14 (1998) 3590.
[27] M. Byloos, H. Al-Maznai, M. Morin, Formation of a self-assembled
monolayer via the electrospreading of physisorbed micelles of thiolates,
J. Phys. Chem. B 103 (1999) 6554.
[28] A. Bad´ıa, Asymptotic theory for the inverse problem in magnetic force
microscopy of superconductors, Phys. Rev. B 60 (1999) 10436.
[29] S.-S. Wong, M. D. Porter, Origin of the multiple voltammetric des-
orption waves of long-chain alkanethiolate monolayers chemisorbed on
annealed gold electrodes, J. Electroanal. Chem. 485 (2000) 135.
[30] H. Wano, K. Uosaki, In situ, real-time monitoring of the reductive des-
orption process of self-assembled monolayers of hexanethiol on Au(111)
surfaces in acidic and alkaline aqueous solutions by scanning tunneling
microscopy, Langmuir 17 (2001) 8224.
[31] C. Vericat, G. Andreasen, M. E. Vela, H. Martin, R. C. Salvarezza,
Following transformation in self-assembled alkanethiol monolayers on
Au(111) by in situ scanning tunneling microscopy, J. Chem. Phys. 115
(2001) 6672.
[32] Y.-T. Long, H.-T. Rong, M. Buck, M. Grunze, Odd-even effects in
the cyclic voltammetry of self-assembled monolayers of biphenyl based
thiols, J. Electroanal. Chem. 524 (2002) 62.
[33] T. Kakiuchi, H. Usui, D. Hobara, M. Yamamoto, Voltammetric proper-
ties of the reductive desorption of alkanethiol self-assembled monolayers
from a metal surface, Langmuir 18 (2002) 5231.
[34] I. Thom, M. Buck, Electrochemical stability of self-assembled monolay-
ers of biphenyl based thiols studied by cyclic voltammetry and second
harmonic generation, Surf. Sci. 581 (2005) 33.
[35] T. Laredo, J. Leitch, M. Chen, I. J. Burgess, J. R. Dutcher, J. Lipkowski,
Measurement of the charge number per adsorbed molecule and packing
densities of self-assembled long-chain monolayers of thiols, Langmuir 23
(2007) 6205.
[36] R. Aguilar-Sanchez, G. J. Su, M. Homberger, U. Simon, T. Wand-
lowski, Structure and electrochemical characterization of 4-methyl-4-
(n-mercaptoalkyl)biphenyls on Au(111)-(1×1), J. Phys. Chem. C 111
(2007) 17409.
[37] I. Thom, M. Buck, On the interpretation of multiple waves in cyclic
voltammograms of self-assembled monolayers of n-alkane thiols on gold,
Z. Phys. Chem. 222 (2008) 739.
[38] X. Cai, S. Baldelli, Surface barrier properties of self-assembled mono-
layers as deduced by sum frequency generation spectroscopy and elec-
trochemistry, J. Phys. Chem. C 115 (2011) 19178.
[39] C. Humbert, B. Busson, C. Six, A. Gayral, M. Gruselle, F. Villain,
A. Tadjeddine, Sum-frequency generation as a vibrational and electronic
probe of the electrochemical interface and thin films, J. Electroanal.
Chem. 621 (2008) 314.
[40] H. Zhang, S. Baldelli, Alkanethiol monolayers at reduced and oxidized
zinc surfaces with corrosion proctection: a sum frequency generation and
electrochemistry investigation, J. Phys. Chem. B 110 (2006) 24062.
[41] H. Zhang, C. Romero, S. Baldelli, Preparation of alkanethiol monolay-
ers on mild steel surfaces studied with sum frequency generation and
electrochemistry, J. Phys. Chem. B 109 (2005) 15520.
[42] Y. Zhang, Y. Tong, M. Abe, K. Uosaki, M. Osawa, Y. Sasaki, S. Ye, Fab-
rication of photochemical pattern on a self-assembled monolayer (SAM)
of a ruthenium cluster under electrochemical control, J. Mater. Chem.
19 (2009) 261.
[43] W. Azzam, A. Bashir, A. Terfort, T. Strunskus, C. W¨oll, Combined
STM and FTIR characterization of terphenylalkanethiol monolayers on
Au(111): Effect of alkyl chain length and deposition temperature, Lang-
muir 22 (2006) 3647.
[44] Z. J. Donhauser, B. A. Mantooth, K. F. Kelly, L. A. Bumm, J. D.
Monnell, J. J. Stapleton, D. W. Price, A. M. Rawlett, D. L. Allara,
J. M. Tour, P. S. Weiss, Conductance switching in single molecules
through conformational changes, Science 292 (2001) 2303.
[45] D. I. Gittins, D. Bethell, D. J. Schiffrin, R. J. Nichols, A nanometre-scale
electronic switch consisting of a metal cluster and redox-addressable
groups, Nature 408 (2000) 67.
[46] M. I. Muglali, A. Bashir, A. Terfort, M. Rohwerder, Electrochemi-
cal investigations on stability and protonation behavior of pyridine-
terminated aromatic self-assembled monolayers, Phys. Chem. Chem.
Phys. 13 (2011) 15530.
[47] B. Schupbach, A. Terfort, A divergent synthesis of oligoarylalkanethiols
with lewis-basic n-donor termini, Org. Biomol. Chem. 8 (2010) 3552.
[48] Y. Chen, P. Schneider, A. Erbe, Investigation of native oxide growth
on zinc in different atmospheres by spectroscopic ellipsometry, Phys.
Status Solidi A 209 (2012) 846.
[49] Y. Chen, A. Erbe, In situ spectroscopic ellipsometry during electro-
chemical treatment of zinc in alkaline carbonate electrolyte, Surf. Sci.
607 (2013) 39.
[50] R. M. A. Azzam, N. M. Bashara, Ellipsometry and Polarized Light,
Elsevier Science, Amsterdam, 1999.
[51] J. Lekner, Theory of Reflection of Electromagnetic and Particle Waves,
M. Nijhoff, Dordrecht, 1987.
[52] D. R. Lide (Ed.), Handbook of Chemistry and Physics, CRC Press, Boca
Raton, 90th edition, 2009.
[53] P. G. Etchegoin, E. C. Le Ru, M. Meyer, An analytic model for the
optical properties of gold, J. Chem. Phys. 125 (2006) 164705.
[54] P. G. Etchegoin, E. C. L. Ru, M. Meyer, Erratum: "An analytic model
for the optical properties of gold" [J. Chem. Phys., 164705 (2006)], J.
Chem. Phys. 127 (2007) 189901.
[55] D. Verreault, V. Kurz, C. Howell, P. Koelsch, Sample cells for probing
solid/liquid interfaces with broadband sum-frequency-generation spec-
troscopy, Rev. Sci. Instrum. 81 (2010) 063111.
[56] A. Lagutchev, S. A. Hambir, D. D. Dlott, Nonresonant background
suppression in broadband vibrational sum-frequency generation spec-
troscopy, J. Phys. Chem. C 111 (2007) 13645.
[57] J. Liu, B. Schupbach, A. Bashir, O. Shekhah, A. Nefedov, M. Kind,
A. Terfort, C. W¨oll, Structural characterization of self-assembled mono-
layers of pyridine-terminated thiolates on gold, Phys. Chem. Chem.
Phys. 12 (2010) 4459.
[58] D.-F. Yang, M. Morin, Chronoamperometric study of the reduction of
chemisorbed thiols on Au(111), J. Electroanal. Chem. 429 (1997) 1.
[59] D.-F. Yang, M. Morin, Chronoamperometric study of the reductive des-
orption of alkanethiol self-assembled monolayers, J. Electroanal. Chem.
441 (1998) 173.
[60] K. Arihara, T. Ariga, N. Takashima, K. Arihara, T. Okajima, F. Ki-
tamura, K. Tokuda, T. Ohsaka, Multiple voltammetric waves for re-
ductive desorption of cysteine and 4-mercaptobenzoic acid monolayers
self-assembled on gold substrates, Phys. Chem. Chem. Phys. 5 (2003)
[61] M. S. El-Deab, K. Arihara, T. Ohsaka, Fabrication of Au(111)-like poly-
crystalline gold electrodes and their applications to oxygen reduction,
J. Electrochem. Soc. 151 (2004) E213.
no, J. Sevilla, T. Pineda, A. Rom´an, M. Bl´azquez, A voltam-
metric study of 6-mercaptopurine monolayers on polycrystalline gold
electrodes, J. Electroanal. Chem. 506 (2001) 92.
[63] J. Strutwolf, C. O'Sullivan, Microstructures by selective desorption of
self-assembled monolayer from polycrystalline gold electrodes, Electro-
analysis 19 (2007) 1467.
[64] T. Kondo, T. Sumi, K. Uosaki, A rotating gold ring-gold disk electrode
study on electrochemical reductive desorption and oxidative readsorp-
tion of a self-assembled monolayer of dodecanethiol, J. Electroanal.
Chem. 538 (2002) 59.
[65] P. Koelsch, M. Muglali, M. Rohwerder, A. Erbe, Third-order effects
in resonant sum-frequency-generation signals at electrified metal/liquid
interfaces, J. Opt. Soc. Am. B accepted (2012).
[66] D.-F. Yang, H. Al-Maznai, M. Morin, Vibrational study of the fast
reductive and the slow oxidative desorptions of a nonanethiol self-
assembled monolayer from a Au(111) single crystal electrode, J. Phys.
Chem. B 101 (1997) 1158.
[67] M. Esplandiu, M. Carot, F. Cometto, V. Macagno, E. Patrito,
Electrochemical STM investigation of 1,8-octanedithiol monolayers on
Au(111).: Experimental and theoretical study, Surf. Sci. 600 (2006) 155.
[68] J. N. Israelachvili, Intermolecular and Surface Forces, Academic Press,
London, 1992.
[69] E. Torres, P. U. Biedermann, A. T. Blumenau, The role of gold adatoms
in self-assembled monolayers of thiol on Au(111), Int. J. Quantum
Chem. 109 (2009) 3466.
[70] D. P. Woodruff, The interface structure of n-alkylthiolate self-assembled
monolayers on coinage metal surfaces, Phys. Chem. Chem. Phys. 10
(2008) 7211.
[71] H. Hakkinen, The gold-sulfur interface at the nanoscale, Nat. Chem. 4
[72] H. Wano, K. Uosaki, In situ dynamic monitoring of electrochemical ox-
idative adsorption and reductive desorption processes of a self-assembled
monolayer of hexanethiol on a Au(111) surface in KOH ethanol solution
by scanning tunneling microscopy, Langmuir 21 (2005) 4024.
[73] D. Hobara, M. Yamamoto, T. Kakiuchi, Reconstruction of Au(111)
following the reductive desorption of self-assembled monolayers of 2-
mercaptoethanesulfonic acid studied by in situ scanning tunneling mi-
croscopy, Chem. Lett. 30 (2001) 374.
[74] D. Hobara, M. Yamamoto, T. Kakiuchi, Reconstruction of Au(111)
following the reductive desorption of self-assembled monolayers of 2-
mercaptoethanesulfonic acid studied by in situ scanning tunneling mi-
croscopy (pg 375, 2001), Chem. Lett. 30 (2001) 1200.
[75] I. Burgess, V. Zamlynny, G. Szymanski, A. Schwan, R. Faragher, J. Lip-
kowski, J. Majewski, S. Satija, Neutron reflectivity studies of field driven
transformations in a monolayer of 4-pentadecyl pyridine at Au electrode
surfaces, J. Electroanal. Chem. 550 (2003) 187.
[76] I. Burgess, M. Li, S. Horswell, G. Szymanski, J. Lipkowski, J. Majewski,
S. Satija, Electric field-driven transformations of a supported model
biological membrane - an electrochemical and neutron reflectivity study,
Biophys. J. 86 (2004) 1763.
[77] D.-F. Yang, C. P. Wilde, M. Morin, Studies of the electrochemical
removal and efficient re-formation of a monolayer of hexadecanethiol self-
assembled at an Au(111) single crystal in aqueous solutions, Langmuir
13 (1997) 243.
[78] M. Byloos, S. Rifai, H. Al-Maznai, M. Laferri ere, M. Morin, A second
harmonic generation study of a physisorbed precursor to the electrode-
position of a monolayer of alkanethiols, Langmuir 17 (2001) 2478.
[79] M. M. Kreevoy, E. T. Harper, R. E. Duvall, H. S. Wilgus, L. T. Ditsch,
Inductive effects on the acid dissociation constants of mercaptans, J.
Am. Chem. Soc. 82 (1960) 4899.
• Araliphatic SAM with extraordinary electrochemical stability
• Enhancement of the hydrogen evolution after reductive SAM desorp-
• In situ real time sum frequency generation spectroscopy (SFG)
• Relation between reductive SAM desorption and hydrogen evolution
Graphical abstract
Source: http://www.mpie.de/3254517/FinalSubmittedVersion__ElectrochimActa__2012__Muglali_Title.pdf
Getränke Havanna vo „Staubfänger"-Phalanx fürs heimatliche Wohnzimmer Havanna von A bis Z Adressen Nummer 169 A an der Ecke der Straßen Virtudes und Amistad. Die Adressen in Havanna sind recht Damit man sich leichter nach dem Weg verwirrend – auf den ersten Blick. Bei erkundigen kann, sind in diesem City- näherer Betrachtung sind sie allerdings
For the use of an Registered Medical Practitioner or a Hospital or a Laboratory only USE IN SPECIAL POPULATIONS Teratogenic EffectsPregnancy Category B: Teratogenicity studies have been performed in animals. Brimonidine tartrate was not teratogenic when given orally Rimoflo ™ Soft during gestation days 6 through 15 in rats and days 6 through 18 in